Structural lesion in brain. Brain Lesions: Understanding Symptoms, Causes, and Treatment Options
What are brain lesions. How do they affect brain function. What causes brain lesions to develop. What are the common symptoms of brain lesions. How are brain lesions diagnosed and treated. Can brain lesions be prevented. What is the long-term outlook for patients with brain lesions.
The Complexity of Brain Lesions: A Comprehensive Overview
Brain lesions represent a significant area of concern in neurological health. These abnormalities in brain tissue can arise from various causes and manifest in diverse symptoms, making them a complex subject of study and treatment. Understanding brain lesions is crucial for both medical professionals and patients alike, as they can have profound impacts on cognitive function, physical abilities, and overall quality of life.
To comprehend brain lesions fully, it’s essential to first grasp the intricate workings of the brain itself. This remarkable organ controls our thoughts, memories, speech, movement, and organ functions. It’s divided into four primary lobes, each with specific roles:
- Frontal lobe: Responsible for motor skills, language, and intellectual and behavioral functions
- Temporal lobe: Crucial for hearing, memory, and speech
- Parietal lobe: Interprets sensory information like heat, pressure, and pain
- Occipital lobe: Primarily responsible for vision
When lesions occur in these areas, they can disrupt normal brain function, leading to a wide array of symptoms and complications.
Unraveling the Causes of Brain Lesions
Brain lesions can develop due to numerous factors, some of which are preventable while others are beyond our control. Understanding these causes is crucial for both prevention and treatment strategies.
Common Causes of Brain Lesions
- Aging
- Genetic predisposition
- Vascular conditions (e.g., stroke, high blood pressure)
- Traumatic brain injuries
- Infections (e.g., meningitis, encephalitis)
- Tumors (primary or metastatic)
- Autoimmune diseases (e.g., lupus, multiple sclerosis)
- Plaques (as seen in Alzheimer’s disease)
- Exposure to radiation or certain chemicals
- Toxins (e.g., excessive alcohol consumption)
- Poor diet
Is there a single most common cause of brain lesions? While many factors contribute to their development, stroke and vascular injuries are perhaps the leading causes. These events can significantly impair blood supply to the brain, resulting in tissue damage and lesion formation.
Recognizing the Symptoms: When to Seek Medical Attention
The symptoms of brain lesions can vary widely depending on their location, size, and underlying cause. Some lesions may be asymptomatic, while others can produce severe and debilitating effects. Recognizing these symptoms is crucial for early detection and treatment.
General Symptoms of Brain Lesions
- Sudden, severe headaches that worsen over time
- Nausea and vomiting
- Impaired movement or coordination
- Difficulty concentrating or making decisions
- Delayed speech, blurred vision, or impaired hearing
- Involuntary movements or convulsions
Are there specific symptoms associated with lesions in different parts of the brain? Indeed, the location of a lesion can produce distinct symptoms:
Frontal Lobe Lesion Symptoms
- Loss of smell (often in one nostril)
- Speech impairment
- Motor activity loss on one or both sides of the body
- Behavioral changes
Temporal Lobe Lesion Symptoms
- Changes in behavior and emotions
- Disruption in smell, taste, and hearing
- Language and speech difficulties
When should you seek medical attention for these symptoms? Any sudden onset of these symptoms, particularly severe headaches or changes in vision, speech, or motor function, warrants immediate medical evaluation.
Diagnostic Approaches: Identifying Brain Lesions
Accurate diagnosis of brain lesions is crucial for determining appropriate treatment strategies. Modern medical technology offers several sophisticated methods for detecting and characterizing these abnormalities.
Common Diagnostic Tools for Brain Lesions
- Magnetic Resonance Imaging (MRI): Provides detailed images of brain structure
- Computed Tomography (CT) scan: Useful for detecting bleeding, swelling, and some tumors
- Positron Emission Tomography (PET) scan: Can reveal brain activity and metabolism
- Electroencephalogram (EEG): Measures electrical activity in the brain
- Lumbar puncture: Analyzes cerebrospinal fluid for signs of infection or inflammation
How do doctors determine which diagnostic tool to use? The choice often depends on the suspected cause of the lesion, the patient’s symptoms, and the need for immediate results. For instance, CT scans are often used in emergency situations due to their speed, while MRIs provide more detailed images for comprehensive evaluations.
Treatment Strategies: Addressing Brain Lesions
Treatment for brain lesions varies widely depending on their cause, location, and severity. A multidisciplinary approach is often necessary, involving neurologists, neurosurgeons, and other specialists.
Common Treatment Approaches
- Medication: To manage symptoms or treat underlying causes
- Surgery: To remove tumors or relieve pressure
- Radiation therapy: For certain types of tumors
- Chemotherapy: For cancerous lesions
- Rehabilitation: To improve function and quality of life
Can all brain lesions be treated? While many lesions can be effectively managed or treated, some may be inoperable or resistant to treatment. In these cases, the focus shifts to symptom management and maintaining quality of life.
Prevention Strategies: Reducing the Risk of Brain Lesions
While not all brain lesions are preventable, certain lifestyle choices and medical interventions can reduce the risk of developing some types of lesions.
Key Prevention Strategies
- Managing high blood pressure and other cardiovascular risk factors
- Wearing protective gear during high-risk activities to prevent head injuries
- Maintaining a healthy diet and exercise regimen
- Limiting alcohol consumption and avoiding tobacco use
- Getting vaccinated against infections that can lead to encephalitis
- Regular medical check-ups, especially for those with a family history of brain lesions
How effective are these prevention strategies? While they can significantly reduce the risk of certain types of lesions, particularly those related to lifestyle factors and vascular health, they cannot eliminate all risk. Genetic factors and some environmental exposures may still contribute to lesion development.
Living with Brain Lesions: Long-term Outlook and Quality of Life
The long-term outlook for individuals with brain lesions can vary greatly depending on the type, location, and severity of the lesion, as well as the effectiveness of treatment. Many people with brain lesions can lead fulfilling lives with proper management and support.
Factors Affecting Long-term Outlook
- Early detection and treatment
- The underlying cause of the lesion
- The affected area of the brain
- The individual’s overall health and age
- Access to comprehensive care and rehabilitation services
What can patients do to improve their quality of life? Engaging in rehabilitation programs, adhering to treatment plans, maintaining a healthy lifestyle, and seeking emotional support can all contribute to better outcomes and improved quality of life for those living with brain lesions.
Advancing Research: The Future of Brain Lesion Treatment
The field of neurology is continuously evolving, with new research offering hope for improved diagnosis, treatment, and prevention of brain lesions. From advanced imaging techniques to innovative therapies, the landscape of brain lesion management is rapidly changing.
Promising Areas of Research
- Gene therapy for genetic causes of brain lesions
- Immunotherapy for treating certain types of tumors
- Stem cell therapy for regenerating damaged brain tissue
- Artificial intelligence in diagnosis and treatment planning
- Targeted drug delivery systems for more effective treatments
How might these advancements change the future of brain lesion treatment? As research progresses, we may see more personalized treatment approaches, earlier detection of lesions, and potentially even ways to reverse some types of brain damage. These advancements could significantly improve outcomes and quality of life for patients with brain lesions.
Brain lesions remain a complex and challenging area of neurological health. However, with ongoing research, improved diagnostic techniques, and advanced treatment options, the outlook for patients with brain lesions continues to improve. By understanding the causes, recognizing the symptoms, and adhering to preventive measures, we can work towards reducing the impact of brain lesions on individuals and society as a whole.
Brain Lesions: Symptoms, Causes, Treatments
Overview
What are brain lesions?
Brain lesions are a type of damage to any part of brain. Lesions can be due to disease, trauma or a birth defect. Sometimes lesions appear in a specific area of the brain. At other times, the lesions are present in a large part of the brain tissue. At first, brain lesions may not produce any symptoms. As lesions worsen with time, the symptoms become more noticeable.
How does the brain work?
The brain controls thoughts, memory, speech, movements of the limbs, and organ function. There are many parts to the brain, and each section has a specific role to play in the human body.
Four lobes make up the brain:
Frontal lobe – the largest of the four lobes, is responsible for the body’s motor skills, such as voluntary movement, language, and intellectual and behavioral functions. This area controls memory, intelligence, concentration, temper and personality.
Temporal lobe – located on each side of the brain at ear level, is important for hearing, memory and speech.
Parietal lobe – at the center of the brain, is where sensory information like heat, pressure and pain is received and interpreted.
Occipital lobe – found at the back of the brain, is primarily responsible for vision.
Symptoms and Causes
What causes brain lesions to develop?
Brain lesions can be caused by many different triggers. The following factors put a person at greater risk to get brain lesions:
- Aging
- Family history of brain lesions. The risk increases if someone else in the family has had the condition.
- Vascular conditions, such as stroke, high blood pressure, and cerebral artery aneurysms
- Trauma to the brain, which can cause internal bleeding. If not remedied, it could lead to death.
- Infections, harmful germs or bacteria in the brain. These can cause diseases like meningitis and encephalitis (both types of swelling (inflammation) of the brain).
- Tumors that either start in the brain (primary tumors) or travel there (metastatic) via blood or lymphatic vessels
- Autoimmune diseases, such as lupus and multiple sclerosis. These result when the body’s antibodies start to attack the body’s own tissues, such as those tissues in the brain.
- Plaques, or excess build-up of abnormal protein in the brain tissues or in the blood vessels, slowing down the supply of blood to the brain, as seen in clogged arteries. Alzheimer’s disease, a condition that affects a person’s memory, thinking and behavior, develops because of plaques in brain tissues. Multiple sclerosis can also cause plaques in the brain secondary to damaged tissue.
- Exposure to radiation or certain chemicals that increase the chance of tumors and lesions in the brain
- Toxins, such as excessive amounts of alcohol or cigarette smoke, in the body.
Other toxic substances are elevated levels of ammonia and urea in the body due to kidney issues (can affect brain function but may not show discrete brain lesions).
- Poor diet, especially eating foods with excess fats and cholesterol
What diseases cause brain lesions?
- Stroke, vascular injury, or impaired supply of blood to the brain is perhaps the leading cause of lesions on the brain.
- Multiple sclerosis, or MS, is a disease where brain lesions are located in multiple sites of the brain. Those suffering from MS have significant problems with motor and sensory functions.
- Lupus, an autoimmune disease, affects almost all systems of the body ranging from skin to heart, liver, muscles and brain. Brain lesions are typically a symptom of this disease.
- Tumors are also a cause of brain lesions and abnormal growth of brain cells.
What are the symptoms of brain lesions?
Symptoms of brain lesions vary depending on the type of lesion, its extent, and where it is found. Everyone is different and symptoms will vary in individual cases. Many lesions, however, may be in areas of the brain that don’t produce symptoms.
Typical symptoms may include:
- Headaches are usually the first symptom to appear with brain lesions. The pain appears suddenly and worsens as time passes. Over-the-counter medicine usually offers no relief for the pain.
- Nausea and possible vomiting
- Impaired movement, if the lesion affects the part of the brain responsible for motor skills
- Lack of concentration, the inability to make quick decisions, and agitation
- Delayed speech, blurred vision, and impaired hearing
- Involuntary movements of body parts, which may progress to convulsions in severe cases
The following symptoms are specific to lesions of the frontal lobe:
- Absence of sense of smell, usually limited to one nostril
- Speech impairment
- Loss of motor activity on one or both sides of the body
- Behavioral changes
The following symptoms are specific to lesions of the temporal lobe:
- A change in behavior and emotions
- Disruption in the sense of smell, taste, and hearing
- Language and speech disorders
- Problems with field of vision
- Forgetfulness and the inability to focus
The following symptoms are specific to lesions of the parietal lobe:
- Loss of sensations like touch
- Astereognosis, or the inability to identity things placed in the hand
- Weakening of language development
The following symptoms are specific to lesions of the occipital lobe:
Diagnosis and Tests
How are brain lesions diagnosed?
If symptoms suggest that a person may be suffering from a brain lesion(s), it is important to contact the doctor for an appointment. A doctor will help diagnose and offer treatment options for each patient depending on the extent of the condition.
The doctor will ask questions about the patient’s symptoms and medical history and then perform a physical examination.
In order to find the location of the lesion, the doctor may touch the patient’s skin with hot, cold or vibrating objects, and also may pinch the patient to check for the feeling of pain. Additional tests may also be recommended by the doctor to further assess the condition.
What tests diagnose brain lesions?
After a physical examination, the doctor may also recommend that the patient schedule a diagnostic test, such as a computed tomography, or CT or CAT scan, or magnetic resonance imaging, or MRI. These tests will help the doctor pinpoint the location of the lesion and will also help assess the extent of damage the lesion has caused the brain.
Computed tomography (CT or CAT scan) is a diagnostic image used to evaluate bone, blood and brain tissue. Sometimes, a medication is injected through the patient’s vein to help highlight brain structures. A CT scan uses radiation.
Magnetic resonance imaging (MRI) is a diagnostic test that produces three-dimensional, or 3D, images of the inside of the body using magnetic fields and computer technology. It shows brain tissue detail as well as the brain stem, and cerebellum (posterior brain) better than a CT scan. An MRI of the brain can help determine whether there are signs of prior mini-strokes. A medication (contrast) can also be injected to help high light structures.
Management and Treatment
How are brain lesions treated?
Treatment varies in each individual case depending on the type of lesion, its location, and cause. It is important that a thorough examination be completed by a doctor to develop the appropriate treatment plan.
The treatment options depend on the type of lesions and severity of symptoms. Usually medicines can be used to treat the underlying cause. Surgery may be an option in some cases, such as when the lesions are caused by a brain tumor.
Sometimes, lesions and symptoms don’t improve even after appropriate diagnosis and proper treatment and the goal is to manage symptoms.
Uncovering the hidden side of brain lesions
Much of our existing knowledge about the workings of the healthy brain comes from studying patients who have suffered brain injuries, for example, due to a stroke. However, despite this, we still have little idea of the impact which brain damage has on the networks of neuronal connections across the whole brain which underlie key cognitive functions. Dr Michel Thiebaut de Schotten and colleagues at the ICM Institute in Paris have developed a software package called BCBtoolkit which can help researchers and clinicians understand the effects of brain damage on brain connections. In future, this could help evolve our understanding of higher brain processes, as well as helping neurologists to predict whether a patient will recover or not from a stroke or other brain injuries.
Example of the pathway, highways of the brain, connecting the front with the posterior part of the brain.
Brain lesions are areas of abnormal tissue that have been damaged due to injury or disease, which can range from being relatively harmless to life-threatening. Clinicians typically identify them as unusual dark or light spots on CT or MRI scans which are different from ordinary brain tissue.
But while conventional neuroimaging analyses can detect the location and structure of a lesion, they tell us little about the wider impact of a particular lesion on the brain’s neuronal circuitry. Understanding this is crucial to being able to quantify the effects of brain damage on the whole brain, as well as exploring the effects of such lesions on a person’s behavioural and cognitive abilities. This could improve our understanding of brain function, and lead to better clinical care for patients suffering from brain damage.
How do brain lesions impact brain function?
Traditionally, if a patient has a lesion in a certain part of their brain, and displays a particular set of symptoms, such as reduced spatial awareness or impaired language production, neurologists deduce that these symptoms are a direct result of functional changes in the visibly damaged area.
Phineas Gage (1823-1860), survived a terrible incident in which his left frontal lobe was blown away by a tamping iron bar. As a result, Gage’s personality changed and he became the index case of acquired ‘sociopathy’. Analyses of the neuronal circuitry impaired by the lesion revealed that areas outside the frontal lobe were also disconnected (chances of disconnection indicated in % in the left panel). Importantly, these areas are classically involved in social cognition including decision-making and emotions (right panel). In sum, these areas may well have contributed to the change of personality shown in Phineas Gage as much as the areas damaged by the passage of the tamping bar.
Studying these patients and what happens when damage occurs to individual brain areas has improved our overall understanding of the brain in healthy individuals over the past two centuries. One famous example is the case of Louis Leborgne, who ultimately spent 21 years in Bicêtre Hospital, Paris, having lost the ability to produce coherent speech despite retaining many other faculties such as intelligence and language comprehension. Upon Leborgne’s death in 1861 at the age of 51, an autopsy revealed a large lesion in the part of his frontal lobe known as the posterior inferior frontal gyrus. This specific region became directly associated with our ability to produce meaningful sounds, and ever since it has remained one of the most widely studied language regions in cognitive psychology.
How variable are the brain connections? Recent results developed by the team of Michel Thiebaut de Schotten, demonstrate that long deep connections of the brain are the most invariant while short connections at the surface of the brain, show a great deal of difference from one person to another.
Through similar case studies, many other high-level cognitive processes such as attention, memory, and problem solving have become associated with different localised brain regions. However, while this may appear to be a logical way to determine how the brain operates, some scientists have long suspected that this has painted a picture of the brain which is rather too simplistic.
The problem is that different neurological processes are not purely confined to individual specialised regions. Instead, many of the brain’s estimated 100 billion neurons form connections between very distant parts of the brain, and it may be that the real impact of brain lesions is through interrupting these complex networks. Disconnections in the brain’s circuitry could have functional and anatomical consequences for brain regions located a long distance away from the lesion. For example, if certain regions are no longer receiving a signal because of a lesion, they can no longer take part in the function they were involved in. This can result in many of the neurons in these regions becoming subject to atrophy, as well as reductions in dendrite and synapse density, and ultimately neuronal death through a programmed cellular mechanism called apoptosis. Because of this, lesions may well have a wider impact in the brain which extends far beyond the visible damage apparent on imaging scans.
Some scientists have long suspected that our current understanding of the brain has painted a picture which is rather too simplistic.
However, there are relatively few technologies available which researchers can utilise to capture and analyse the long-range effects resulting from lesion-induced brain disconnections. Scientists have previously attempted to use a variety of 3D modelling and image analysis techniques for exploring the nonlocal effects of lesions. However few of these methods are open-access, making them inaccessible to many scientists. In addition, much of the research conducted so far has lacked a standardised way of determining what these techniques are actually measuring, and how they should be combined, affecting the reproducibility of these studies.
Artistic impression of the minimalistic understanding of the brain as a mosaic of separated functional areas. However, current research aims at demonstrating that the brain works as an interconnected unity.
A new method for exploring the impact of brain lesions
Dr Michel Thiebaut de Schotten – principal investigator at the Brain Connectivity and Behaviour Lab at the ICM Institute in Paris – and colleagues have developed a new open-access software package called BCBtoolkit which consists of a set of programs for analysing the long-range effects of brain disconnections.
These programs measure the brain circuitry, estimate the subsequent changes within these circuits which have been caused by a particular lesion and use this information to deduce which changes are contributing to the patient’s symptoms.
Part of the development undertaken by the team of Dr Michel Thiebaut de Schotten is the Atlas of Human Brain connection which maps systems of interconnected areas in the brain.
This is important as studies have suggested that the extent of a patient’s ability to recover from a lesion depends on the exact patterns of change induced within their brain circuitry. For example, one 2014 study of 16 stroke patients found that the ability of these patients to recover from speech impairment following a stroke in the left brain hemisphere, depended on the number of connections remaining between the equivalent of Broca’s area and Wernicke’s area – two of the most important regions for language use – in the right hemisphere.
To prove that BCBtoolkit could be clinically useful for studying patients with brain lesions, Thiebaut de Schotten’s lab have used the package to study the brain connections of 37 patients with frontal lobe lesions resulting from a stroke, infection, hematoma or surgical removal of a brain tumour or of an epileptogenic area.
Initially, they applied the software to map the patient’s lesions onto virtual brain scans of hundreds of healthy individuals, showing every neural connection going to and from the damaged areas. These representations enabled them to visualise which brain regions had been structurally disconnected by the lesions.
To understand the link between these disconnections and the patients’ symptoms, they then conducted various neuropsychological assessments on each patient, such as category fluency testing where patients undergo different exercises such as naming as many animals as they can in two minutes.
How variable are the brain connections? Recent results developed by the team of Michel Thiebaut de Schotten, demonstrate that long deep connections of the brain are the most invariant while short connections at the surface of the brain, show a great deal of difference from one person to another.
The results of these tests suggested that the patients were suffering from cognitive problems such as impaired language, working memory and verbal fluency, related to brain disconnections in major networks associated with processes such as executive function, as well as language and semantic production.
Having identified the precise regions affected by brain disconnections in these patients, the researchers used BCBtoolkit to analyse data collected on these regions from MRI scans. By measuring cortical thickness, they were able to estimate the total neuronal loss in these areas. This provided further evidence that many of the patients’ cognitive impairments were at least partially linked to a loss of cortical neurons in regions located far away from the original lesion.
“For the very first time, using our program, we were able to measure disconnections within the brain of a group of patients and associate neuropsychological deficit with disconnection,” Michel Thiebaut de Schotten explained. “We hope to better understand the brain’s underlying mechanisms and increase symptom predictability in patients with brain lesions.”
Brain connections are the highways of the brain conducting information at more than 300km/h.
The future
Patients with brain lesions have always provided researchers with a unique opportunity to understand the functioning of the human mind. Through BCBtoolkit, scientists working in this field now have, for the first time, a scientifically validated set of methods for capturing the impact of brain damage on the whole brain.
This allows scientists to try and discern the natural history of events which occurred in the brain following a lesion, as well as exploring the relationship between these damaged areas and behavioural and cognitive symptoms.
But while this is currently just a research tool, in future it could have considerable clinical benefit for patients themselves. Every year, more than 2 million people across Europe suffer a stroke, often resulting in persistent lesions which affect their personality, quality of life and prevent them from being able to return to work. As a result, there is a considerable clinical need for diagnostic technologies which allow neurologists to inform these patients and their families in a timely manner, the extent to which their symptoms will resolve.
BCBtoolkit allows scientists to discern the natural history of events which occurred in the brain following a lesion.
However, despite decades of scientific studies describing the symptoms resulting from different lesions, neurologists still typically have little idea of how well a patient will recover from a particular lesion. The only option available is simply to observe how that patient progresses over the course of weeks and months.
But through BCBtoolkit and other similar packages being developed for lesion-symptom mapping, this could soon change. By using these techniques to study the impact of different sized lesions in different locations in the brain, neurologists will be able to stratify patient populations more accurately than ever before. This will allow them to predict the patients who are more likely to make a partial or full recovery, enabling them to act much sooner with treatment and rehabilitation plans for patients who have sustained lasting damage. Such knowledge will allow patients and their families to begin making appropriate arrangements with employers and in many countries, health insurance providers, at a much earlier stage than usual, reducing the burden and stress associated with stroke.
Can you describe how neurologists could use BCBtoolkit to predict how well a patient will recover from a particular brain lesion?
Neurologists can use our software to estimate the extent of the brain disconnection. The more the disconnection of the brain, the less likely the patient will recover. We are now implementing new open tools which will not only decode the patient symptoms but also provide a tailor-made indication of potential recovery for any stroke patient no matter where their lesion occurs. We plan to apply the same technology in the future for the planning of brain surgery and the early identification and the stratification of neurodegenerative disorders such as Alzheimer.
What are your future plans for research in this area?
Future plans include understanding how the brain can change its functioning to compensate for impairment and how we can help the brain to better achieve these compensatory changes. Additionally, we wish to understand what the differences are between patients in term of anatomy and functioning of the brain that drives their differences in recovery.
References
- Foulon C, Cerliani L, Kinkingnéhun S, Levy R, Rosso C, Urbanski M, Volle E, Thiebaut de Schotten M.
(2018).‘Advanced lesion symptom mapping analyses and implementation as BCBtoolkit’. Gigascience, 7(3), giy004. PMID: 29432527.
- Thiebaut de Schotten M, Dell’Acqua F, RatiuP,Leslie A, Howells H, Cabanis E, Iba-Zizen MT, Plaisant O, Simmons A, Dronkers NF, Corkin S, Catani M. (2015).‘From Phineas Gage and Monsieur Leborgne to H.M.: Revisiting Disconnection Syndromes’. Cereb Cortex, 25(12),4812–4827. PMID: 26271113.
Right-sided brain lesions predominate among patients with lesional mania: evidence from a systematic review and pooled lesion analysis
Stein, G., Wilkinson, G. Seminars in General Adult Psychiatry (RCPsych Publications, 2007).
Krauthammer, C. & Klerman, G. L. Secondary mania: manic syndromes associated with antecedent physical illness or drugs. Arch. Gen. Psychiatry 35, 1333–1339 (1978).
CAS
PubMed
Google Scholar
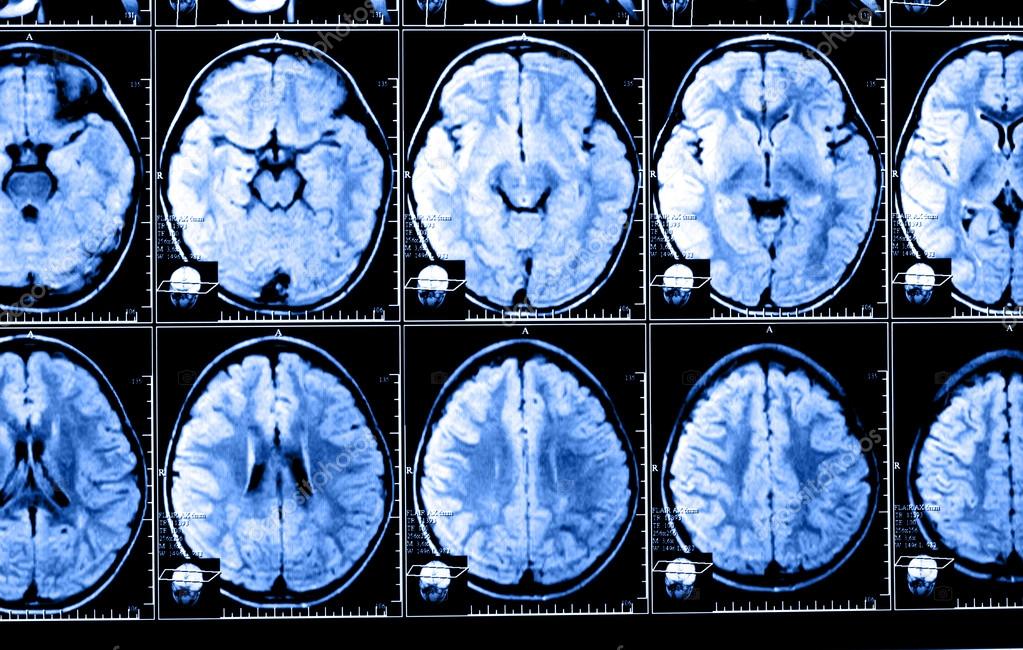
Satzer, D. & Bond, D. J. Mania secondary to focal brain lesions: implications for understanding the functional neuroanatomy of bipolar disorder. Bipolar Disord. 18, 205–220 (2016).
PubMed
Google Scholar
Morken, G., Vaaler, A. E., Folden, G. E., Andreassen, O. A. & Malt, U. F. Age at onset of first episode and time to treatment in in-patients with bipolar disorder. Br. J. Psychiatry 194, 559–560 (2009).
PubMed
Google Scholar
Braun, C. M., Daigneault, R., Gaudelet, S. & Guimond, A. Diagnostic and Statistical Manual of Mental Disorders, Fourth Edition symptoms of mania: which one(s) result(s) more often from right than left hemisphere lesions? Compr. Psychiatry 49, 441–459 (2008).
PubMed
Google Scholar
Cummings, J. L. Organic psychoses: delusional disorders and secondary mania. Psychiatr. Clin. 9, 293–311 (1986).
CAS
Google Scholar
Rorden, C. & Karnath, H.-O. Using human brain lesions to infer function: a relic from a past era in the fMRI age? Nat. Rev. Neurosci. 5, 812–819 (2004).
CAS
Google Scholar
American Psychiatric Association. Diagnostic and Statistical Manual of Mental Disorders (DSM-5®) (American Psychiatric Association Pub., 2013).
Berthier, M. L., Kulisevsky, J., Gironell, A. & Fernandez Benitez, J. A. Poststroke bipolar affective disorder: clinical subtypes, concurrent movement disorders, and anatomical correlates. J. Neuropsychiatry Clin. Neurosci. 8, 160–167 (1996).
CAS
PubMed
Google Scholar
Robinson, R. G., Boston, J. D., Starkstein, S. E. & Price, T. R. Comparison of mania and depression after brain injury: causal factors. Am. J. psychiatry 145, 172–178 (1988).
CAS
PubMed
Google Scholar
Starkstein, S. E., Pearlson, G. D., Boston, J. & Robinson, R. G. Mania after brain injury. A controlled study of causative factors. Arch. Neurol. 44, 1069–1073 (1987).
CAS
PubMed
Google Scholar
Starkstein, S. E., Fedoroff, P., Berthier, M. L. & Robinson, R. G. Manic-depressive and pure manic states after brain lesions. Biol. Psychiatry 29, 149–158 (1991).
CAS
PubMed
Google Scholar
Rosner, B. Fundamentals of Biostatistics 8th edn. (Nelson Education, 2016).
Ostrom, Q. T. et al. CBTRUS statistical report: primary brain and other central nervous system tumors diagnosed in the United States in 2009–2013. Neuro-Oncol. 18(suppl_5), v1–v75 (2016).
Google Scholar
Sperber, C. & Karnath, H. O. Topography of acute stroke in a sample of 439 right brain damaged patients. NeuroImage: Clin. 10, 124–128 (2016).
Google Scholar
Benjamini, Y. & Hochberg, Y. Controlling the false discovery rate: a practical and powerful approach to multiple testing. J. R. Stat. Soc. Ser. B 57, 289–300 (1995).
Alla, P., de Jaureguiberry, J. P., Galzin, M., Gisserot, O. & Jaubert, D. Hemiballism with manic access caused by toxoplasmic abscess in AIDS. Annales de. Med. interne 148, 507–509 (1997).
CAS
Google Scholar
Alpers, B. J. Relation of the hypothalamus to disorders of personality: report of a case. Arch. Neurol. Psychiatry 38, 291–303 (1937).
Google Scholar

Antelmi, E., Fabbri, M., Cretella, L., Guarino, M. & Stracciari, A. Late onset bipolar disorder due to a lacunar state. Behavioural Neurol. 2014, 780742 (2014).
Google Scholar
Asghar-Ali, A. A., Taber, K. H., Hurley, R. A. & Hayman, L. A. Pure neuropsychiatric presentation of multiple sclerosis. Am. J. Psychiatry 161, 226–231 (2004).
PubMed
Google Scholar
Avery, T. Seven cases of frontal tumour with psychiatric presentation. Br. J. Psychiatry 119, 19–23 (1971).
CAS
PubMed
Google Scholar
Bakchine, S. et al. Manic-like state after bilateral orbitofrontal and right temporoparietal injury: efficacy of clonidine. Neurology 39, 777–781 (1989).
CAS
PubMed
Google Scholar

Bamrah, J. S. & Johnson, J. Bipolar affective disorder following head injury. Br. J. Psychiatry 158, 117–119 (1991).
CAS
PubMed
Google Scholar
Barczak, P., Edmunds, E. & Betts, T. Hypomania following complex partial seizures. A report of three cases. Br. J. Psychiatry 152, 137–139 (1988).
CAS
PubMed
Google Scholar
Belli, H., Akbudak, M., Ural, C. & Kulacaoglu, F. Solitary lesion in ponto-mesencephalic area related secondary mania: a case report. Psychiatr. Danubina. 24, 223–225 (2012).
Google Scholar
Bengesser, S. A. et al. Poststroke-bipolar affective disorder. Fortschr. der Neurologie-Psychiatr. 81, 459–463 (2013).
CAS
Google Scholar
Benjamin, S. , Kirsch, D., Visscher, T., Ozbayrak, K. R. & Weaver, J. P. Hypomania from left frontal AVM resection. Neurology 54, 1389–1390 (2000).
CAS
PubMed
Google Scholar
Benke, T., Kurzthaler, I., Schmidauer, C., Moncayo, R. & Donnemiller, E. Mania caused by a diencephalic lesion. Neuropsychologia 40, 245–252 (2002).
PubMed
Google Scholar
Berthier, M. Post-stroke rapid cycling bipolar affective disorder. Br. J. Psychiatry 160, 283–283 (1992).
CAS
PubMed
Google Scholar
Bhanji, S., Gardner-Thorpe, C. & Rahavard, F. Aqueduct stenosis and manic depressive psychosis. J. Neurol. Neurosurg. Psychiatry 46, 1158–1159 (1983).
CAS
PubMed
PubMed Central
Google Scholar
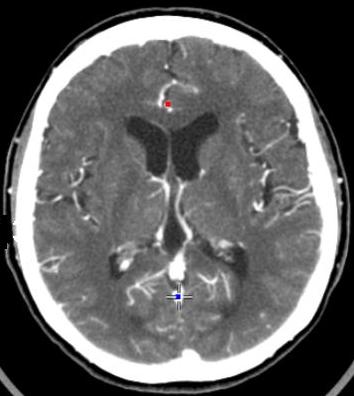
Bhatia, M. S., Srivastava, S., Jhanjee, A. & Oberoi, A. Colloid cyst presenting as recurrent mania. J. Neuropsychiatry Clin. Neurosci. 25, E01–E02 (2013).
PubMed
Google Scholar
Binder, R. L. Neurologically silent brain tumors in psychiatric hospital admissions: three cases and a review. J. Clin. psychiatry 44, 94–97 (1983).
CAS
PubMed
Google Scholar
Bobo, W. V., Murphy, M. J. & Heckers, S. H. Recurring episodes of Bell’s mania after cerebrovascular accident. Psychosomatics 50, 285–288 (2009).
PubMed
Google Scholar
Bogousslavsky, J. et al. Manic delirium and frontal-like syndrome with paramedian infarction of the right thalamus. J. Neurol. Neurosurg. Psychiatry 51, 116–119 (1988).
CAS
PubMed
PubMed Central
Google Scholar

Bornke, C., Postert, T., Przuntek, H. & Buttner, T. Acute mania due to a right hemisphere infarction. Eur. J. Neurol. 5, 407–409 (1998).
Google Scholar
Brooks, J. O. III & Hoblyn, J. C. Secondary mania in older adults. Am. J. Psychiatry 162, 2033–2038 (2005).
PubMed
Google Scholar
Caeiro, L., Ferro, J., Albuquerque, R. & Figueira, M. Mania no AVC agudo. Sinapse 2, 90 (2002).
Google Scholar
Caeiro, L., Santos, C. O., Ferro, J. M. & Figueira, M. L. Neuropsychiatric disturbances in acute subarachnoid haemorrhage. Eur. J. Neurol. 18, 857–864 (2011).
CAS
PubMed
Google Scholar
Calo, J. J. P. et al. Mania after traumatic brain injury – a report of 2 cases and 194 literature-review. Arch. De. Neurobiol. 57, 194–201 (1994).
Google Scholar
Camden, J. R. & Spiegel, D. R. Manic behavior resulting from left frontal closed head injury in an adult with fetal alcohol syndrome. Psychosomatics 48, 433–435 (2007).
PubMed
Google Scholar
Carran, M. A., Kohler, C. G., O’Connor, M. J., Bilker, W. B. & Sperling, M. R. Mania following temporal lobectomy. Neurology 61, 770–774 (2003).
CAS
PubMed
Google Scholar
Celik, Y., Erdogan, E., Tuglu, C. & Utku, U. Post-stroke mania in late life due to right temporoparietal infarction. Psychiatry Clin. Neurosci. 58, 446–447 (2004).
PubMed
Google Scholar
Chimowitz, M. & Furlan, A. Resolution of psychotic depression after right temporoparietal infarction. J. Nerv. Ment. Dis. 178, 458–459 (1990).
CAS
PubMed
Google Scholar
Clark, A. F. & Davison, K. Mania following head injury. A report of two cases and a review of the literature. Br. J. Psychiatry 150, 841–844 (1987).
CAS
PubMed
Google Scholar
Claude, H., Baruk, H., Lamache, A. & Cuel, J. Manic excitation and cerebral tumor. Encephale Rev. Psychiatr. Clin. Biol. Ther. 23, 9–19 (1928).
Google Scholar
Cohen, M. R. & Niska, R. W. Localized right cerebral hemisphere dysfunction and recurrent mania. Am. J. Psychiatry 137, 847–848 (1980).
CAS
PubMed
Google Scholar
Danel, T. et al. Mood disorders and right hemisphere infarction. L’Encephale 15, 549–553 (1989).
CAS
PubMed
Google Scholar
Daniels, J. P. & Felde, A. Quetiapine treatment for mania secondary to brain injury in 2 patients. J. Clin. Psychiatry 69, 497–498 (2008).
PubMed
Google Scholar
Das, P., Chopra, A., Rai, A. & Kuppuswamy, P. S. Late-onset recurrent mania as a manifestation of Wallenberg syndrome: a case report and review of the literature. Bipolar Disord. 17, 677–682 (2015).
PubMed
Google Scholar
Dauncey, K. Mania in the early stages of AIDS. Br. J. Psychiatry 152, 716–717 (1988).
CAS
PubMed
Google Scholar
Drake, M. E. Jr., Pakalnis, A. & Phillips, B. Secondary mania after ventral pontine infarction. J. neuropsychiatry Clin. Neurosci. 2, 322–325 (1990).
PubMed
Google Scholar
El Hechmi, S., Ben Romdhane, I., Belkhiria, A., Medini, F. & Labbene, R. Bipolar disorder in the aftermath of a traumatic brain injury: report of a case. Eur. Psychiatry 28, 54–54 (2013).
Google Scholar
Estrade, J. F. & Samuel-Lajeunesse, B. Secondary mania. Diagnostic problems (apropos of a case of secondary mania in partial complex epilepsy crisis). Ann. Med. Psychol. 147, 662–667 (1989).
CAS
Google Scholar
Fawcett, R. G. Cerebral infarct presenting as mania. J. Clin. Psychiatry 52, 352–353 (1991).
CAS
PubMed
Google Scholar
Fenn, D. & George, K. Post-stroke mania late in life involving the left hemisphere. Aust. NZ J. Psychiatry 33, 598–600 (1999).
CAS
Google Scholar
Filley, C. M. & Kleinschmidt-DeMasters, B. K. Neurobehavioral presentations of brain neoplasms. West. J. Med. 163, 19–25 (1995).
CAS
PubMed
PubMed Central
Google Scholar
Gafoor, R. & O’Keane, V. Three case reports of secondary mania: evidence supporting a right frontotemporal locus. Eur. Psychiatry 18, 32–33 (2003).
PubMed
Google Scholar
Gal, P. Mental symptoms in cases of tumor of temporal lobe. Am. J. Psychiatry 115, 157–160 (1958).
CAS
PubMed
Google Scholar
Galindo Menendez, A. [Parenchymal neurosyphilis. Insidious onset (dementia) and acute onset (manic type) forms]. Actas Luso Esp. Neurol. Psiquiatr. Cienc. Afines 24, 261–267 (1996).
CAS
PubMed
Google Scholar
Garland, E. J. & Zis, A. P. Multiple-sclerosis and affective-disorders. Can. J. Psychiatry Rev. 36, 112–117 (1991).
CAS
Google Scholar
Goyal, R., Sameer, M. & Chandrasekaran, R. Mania secondary to right-sided stroke-responsive to olanzapine. Gen. Hosp. Psychiatry 28, 262–263 (2006).
PubMed
Google Scholar
Greenberg, D. B. & Brown, G. L. Mania resulting from brain stem tumor. J. Nerv. Ment. Dis. 173, 434–436 (1985).
CAS
PubMed
Google Scholar
Haq, M. Z., Dubey, I., Khess, C. R., Das, U. & Kumar, R. Bipolar disorder and tuberous sclerosis complex: is it a mere coincidence? CNS Spectr. 14, 643–647 (2009).
PubMed
Google Scholar
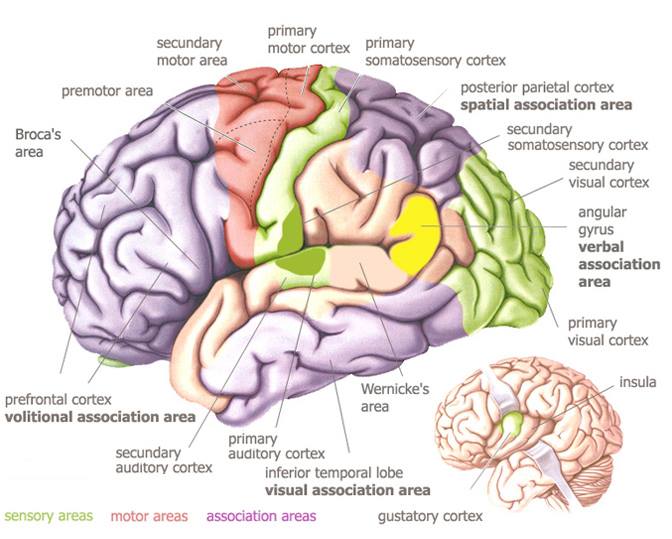
Heinrich, T. W. & Junig, J. T. Recurrent mania associated with repeated brain injury. Gen. Hosp. Psychiatry 26, 490–492 (2004).
PubMed
Google Scholar
Huffman, J. & Stern, T. A. Acute psychiatric manifestations of stroke: a clinical case conference. Psychosomatics 44, 65–75 (2003).
PubMed
Google Scholar
Hunt, N. & Silverstone, T. Seasonal affective disorder following brain injury. Br. J. Psychiatry.: J. Ment. Sci. 156, 884–886 (1990).
CAS
Google Scholar
Inzelberg, R., Nisipeanu, P., Joel, D., Sarkantyus, M. & Carasso, R. L. Acute mania and hemichorea. Clin. Neuropharmacol. 24, 300–303 (2001).
CAS
PubMed
Google Scholar
Isles, L. J. & Orrell, M. W. Secondary mania after open-heart-surgery. Br. J. Psychiatry 159, 280–282 (1991).
CAS
PubMed
Google Scholar
Jagadesan, V., Thiruvengadam, K. R. & Muralidharan, R. Cerebellar Stroke-manifesting as Mania. Indian J. Psychol. Med. 36, 338–340 (2014).
PubMed
PubMed Central
Google Scholar
Jamieson, R. C. & Wells, C. E. Manic psychosis in a patient with multiple metastatic brain tumors. J. Clin. Psychiatry 40, 280–283 (1979).
CAS
PubMed
Google Scholar
Jampala, V. C. & Abrams, R. Mania secondary to left and right hemisphere damage. Am. J. Psychiatry 140, 1197–1199 (1983).
CAS
PubMed
Google Scholar
Julayanont, P., Ruthirago, D., Alam, K. & Alderazi, Y. J. Behavioral Disconnection Syndrome Manifesting as Combined Mania and Visual-Auditory Hallucinations Secondary to Isolated Right Thalamic Hemorrhage. J. Neuropsychiatry Clin. Neurosci. 29, 401–408 (2017).
PubMed
Google Scholar
Kanemoto, K. Hypomania after temporal lobectomy: a sequela to the increased excitability of the residual temporal lobe? J. Neurol., Neurosurg. Psychiatry 59, 448–449 (1995).
CAS
Google Scholar
Kar, S. K., Das, K. K., Jaiswal, A. K. & Jaiswal, S. Mood disorder as an early presentation of epidermoid of quadrigeminal cistern. J. Neurosci. Rural Pract. 8, 443–445 (2017).
PubMed
PubMed Central
Google Scholar
Koreki, A., Takahata, K., Tabuchi, H. & Kato, M. Increased left anterior insular and inferior prefrontal activity in post-stroke mania. BMC Neurol. 12, 68 (2012).
PubMed
PubMed Central
Google Scholar
Kotrla, K. J., Chacko, R. C. & Barrett, S. A. A case of organic mania associated with open heart surgery. J. Geriatr. Psychiatry Neurol. 7, 8–12 (1994).
CAS
PubMed
Google Scholar
Ku, B. D. et al. Secondary mania in a patient with delayed anoxic encephalopathy after carbon monoxide intoxication. J. Clin. Neurosci. 13, 860–862 (2006).
PubMed
Google Scholar
Kulisevsky, J., Berthier, M. L. & Pujol, J. Hemiballismus and secondary mania following a right thalamic infarction. Neurology 43, 1422–1424 (1993).
CAS
PubMed
Google Scholar
Kumar, S. K. & Mahr, G. CADASIL presenting as bipolar disorder. Psychosomatics 38, 397–398 (1997).
CAS
PubMed
Google Scholar
Lauterbach, E. C. Bipolar disorders, dystonia, and compulsion after dysfunction of the cerebellum, dentatorubrothalamic tract, and substantia nigra. Biol. Psychiatry 40, 726–730 (1996).
CAS
PubMed
Google Scholar
Lee, Y. M. Secondary mania in a patient with solitary red nucleus lesion. Psychiatry Clin. Neurosci. 68, 243 (2014).
PubMed
Google Scholar
Leibson, E. Anosognosia and mania associated with right thalamic haemorrhage. J. Neurol., Neurosurg., Psychiatry 68, 107–108 (2000).
CAS
Google Scholar
Liu, C. Y., Wang, S. J., Fuh, J. L., Yang, Y. Y. & Liu, H. C. Bipolar disorder following a stroke involving the left hemisphere. Aust. NZ J. Psychiatry 30, 688–691 (1996).
CAS
Google Scholar
Lupo, M. et al. Evidence of cerebellar involvement in the onset of a manic state. Front. Neurol. 9, 774 (2018).
PubMed
PubMed Central
Google Scholar
Malamud, N. Psychiatric disorder with intracranial tumors of limbic system. Arch. Neurol. 17, 113–123 (1967).
CAS
PubMed
Google Scholar
Mark, M., Modai, I., Aizenberg, D., Heilbronn, Y. & Elizur, A. Bipolar disorder associated with an acoustic neurinoma. Psychiatr. Serv. 42, 1258–1260 (1991).
CAS
Google Scholar
McKeown, S. P. & Jani, C. J. Mania following head injury. Br. J. Psychiatry 151, 867–868 (1987).
CAS
PubMed
Google Scholar
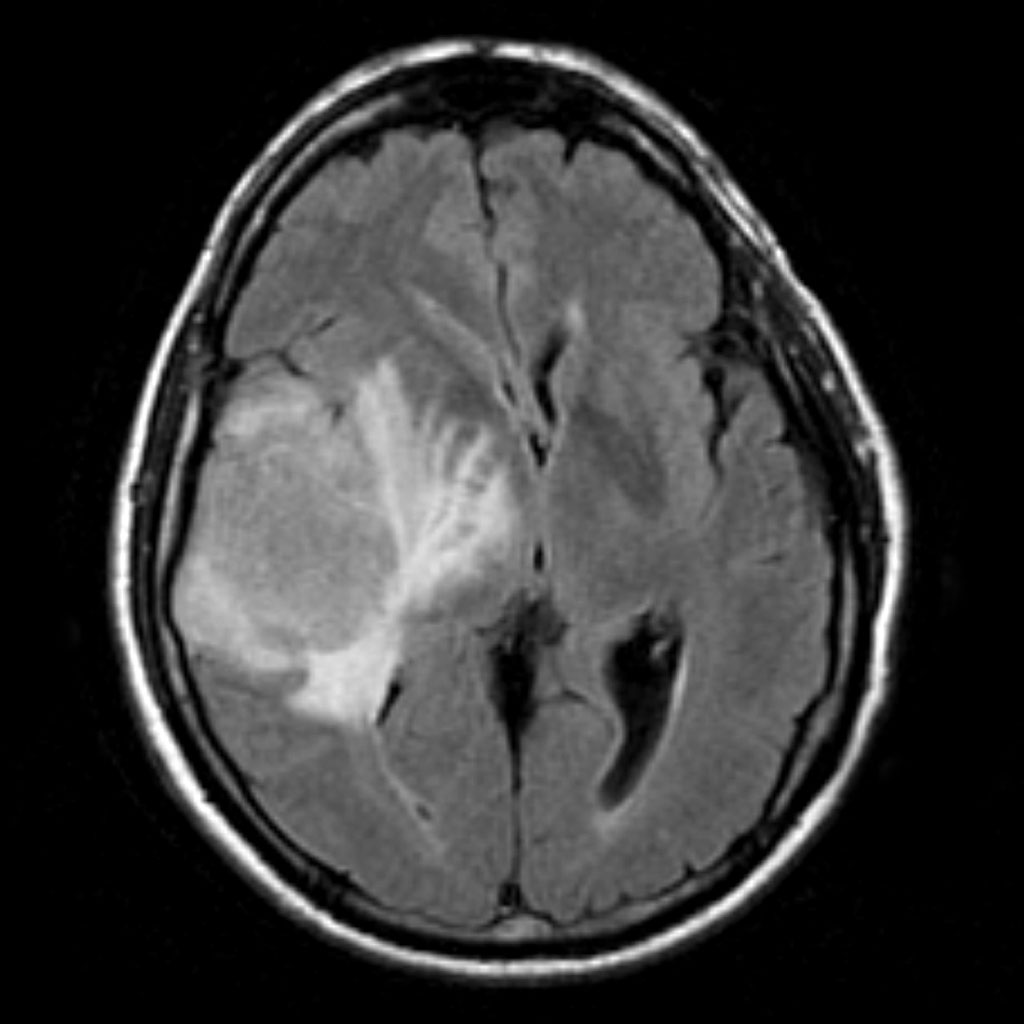
Miller, B. L., Cummings, J. L., McIntyre, H., Ebers, G. & Grode, M. Hypersexuality or altered sexual preference following brain injury. J. Neurol. Neurosurg. Psychiatry 49, 867–873 (1986).
CAS
PubMed
PubMed Central
Google Scholar
Modrego, P. J. & Ferrandez, J. Familial multiple sclerosis with repetitive relapses of manic psychosis in two patients (mother and daughter). Behavioural Neurol. 12, 175–179 (2000).
Google Scholar
Mumoli, N., Pulera, F., Vitale, J. & Camaiti, A. Frontal lobe syndrome caused by a giant meningioma presenting as depression and bipolar disorder. Singap. Med. J. 54, e158–e159 (2013).
Google Scholar
Murai, T. & Fujimoto, S. Rapid cycling bipolar disorder after left temporal polar damage. Brain Inj. 17, 355–358 (2003).
PubMed
Google Scholar
Mustafa, B., Evrim, O. & Sari, A. Secondary mania following traumatic brain injury. J. Neuropsychiatry Clin. Neurosci. 17, 122–124 (2005).
PubMed
Google Scholar
Nagaratnam, N., Tse, A., Lim, R. & Chowdhury, M. Aberrant sexual behaviour following stroke. Eur. J. Intern. Med. 9, 207–210 (1998).
Google Scholar
Nagaratnam, N., Wong, K. K. & Patel, I. Secondary mania of vascular origin in elderly patients: a report of two clinical cases. Arch. Gerontol. Geriatrics 43, 223–232 (2006).
Google Scholar
Nizamie, S. H., Nizamie, A., Borde, M. & Sharma, S. Mania following head injury: case reports and neuropsychological findings. Acta Psychiatr. Scandinavica 77, 637–639 (1988).
CAS
Google Scholar
Koh, O. H., Azreen, H. N., Gill, J. S. & Pillai, S. K. A case of post-stroke mannia. Malays. J. Psychiatry 19, 41–45 (2010).
Google Scholar
Okun, M. S., Bakay, R. A., DeLong, M. R. & Vitek, J. L. Transient manic behavior after pallidotomy. Brain Cogn. 52, 281–283 (2003).
PubMed
Google Scholar
Oppler, W. Manic psychosis in a case of parasagittal meningioma. Arch. Neurol. Psychiatry 64, 417–430 (1950).
CAS
PubMed
Google Scholar
Park, S., Park, B., Koh, M. K. & Joo, Y. H. Case report: bipolar disorder as the first manifestation of CADASIL. BMC Psychiatry 14, 175 (2014).
PubMed
PubMed Central
Google Scholar
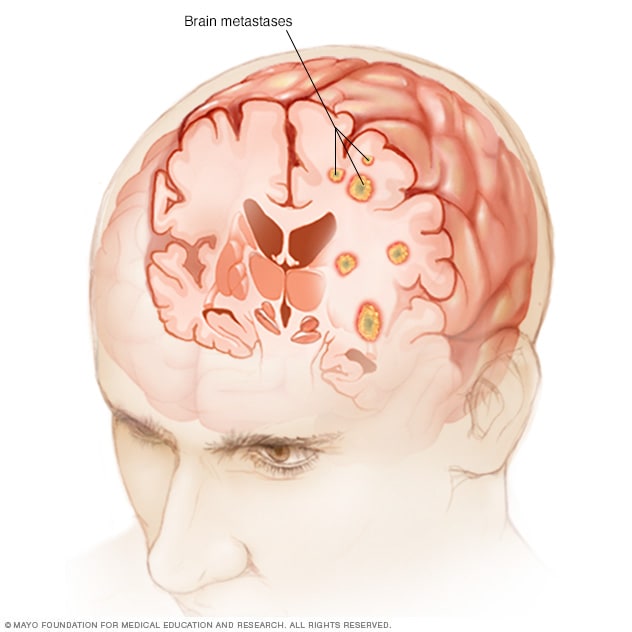
Pathak, A. & Srivastava, M. Post-stroke mania–a case report. Asian J. Psychiatry 15, 209–212 (2014).
Google Scholar
Reisch, T., Brekenfeld, C. & Barth, A. A case of hydrocephalus occlusus presenting as bipolar disorder. Acta Psychiatr. Scand. 112, 159–162 (2005).
CAS
PubMed
Google Scholar
Robinson, R. G., Kubos, K. L., Starr, L., Rao, K. & Price, T. Mood disorders in stroke patients. Brain 107(Pt 1), 81–93 (1984).
PubMed
Google Scholar
Rocha, F. F., Carneiro, J. G., Pereira Pde, A., Correa, H. & Teixeira, A. L. Poststroke manic symptoms: an unusual neuropsychiatric condition. Rev. Bras. Psiquiatr. 30, 173–174 (2008).
PubMed
Google Scholar
Rocha, F. F., Correa, H. & Teixeira, A. L. A successful outcome with valproic acid in a case of mania secondary to stroke of the right frontal lobe. Prog. Neuropsychopharmacol. Biol. Psychiatry 32, 587–588 (2008).
PubMed
Google Scholar
Rosenbaum, A. H. & Barry, M. J. Jr. Positive therapeutic response to lithium in hypomania secondary to organic brain syndrome. Am. J. Psychiatry 132, 1072–1073 (1975).
CAS
PubMed
Google Scholar
Routh, R. & Hill, A. Post‐stroke mania: a rare but treatable presentation. Prog. Neurol. Psychiatry 18, 24–25 (2014).
Google Scholar
Salazar-Calderon Perriggo, V. H., Oommen, K. J. & Sobonya, R. E. Silent solitary right parietal chondroma resulting in secondary mania. Clin. Neuropathol. 12, 325–329 (1993).
CAS
PubMed
Google Scholar
Sanders, R. D. & MathewsT. A. Hypergraphia and Secondary Mania in Temporal Lobe Epilepsy: Case Reports and Literature Review. Cogn. Behav. Neurol. 7, 114–117 (1994).
Google Scholar
Semiz, M., Kavakci, O., Yontar, G. & Yildirim, O. Case of organic mania associated with stroke and open heart surgery. Psychiatry Clin. Neurosci. 64, 587 (2010).
PubMed
Google Scholar
Semiz, U. B. et al. Leptospirosis presenting with mania and psychosis: four consecutive cases seen in a military hospital in Turkey. Int. J. Psychiatry Med. 35, 299–305 (2005).
PubMed
Google Scholar
Sidhom, Y. et al. Bipolar disorder and multiple sclerosis: a case series. Behav. Neurol. 2014, 536503 (2014).
Starkstein, S. E., Boston, J. D. & Robinson, R. G. Mechanisms of mania after brain injury. 12 case reports and review of the literature. J. Nerv. Ment. Dis. 176, 87–100 (1988).
CAS
PubMed
Google Scholar
Starkstein, S. E. et al. Mania after brain injury: neuroradiological and metabolic findings. Ann. Neurol. 27, 652–659 (1990).
CAS
PubMed
Google Scholar
Stern, K. & Dancey, T. E. Glioma of the diencephalon in a manic patient. Am. J. Psychiatry 98, 716–719 (1942).
Google Scholar
Sullivan, G. & Jenkins, P. L. Secondary mania following cerebral hypoxia. Ir. J. Psychological Med. 12, 68–69 (1995).
Google Scholar

Sweet, RA. Case of craniopharyngioma in late life. J. Neuropsychiatry Clin. Neurosci. 2, 464–465 (1990).
CAS
PubMed
Google Scholar
Taylor, J. B., Prager, L. M., Quijije, N. V. & Schaefer, P. W. Case 21-2018: a 61-year-old man with grandiosity, impulsivity, and decreased sleep. N. Engl. J. Med. 379, 182–189 (2018).
PubMed
Google Scholar
Topcuoglu, V., Gimzal Gonentur, A., Bilgin Topcuoglu, O., Yazgan, C. & Kora, K. Mood disorder due to herpes simplex encephalitis with neuroimaging findings limited to the right hemisphere and cerebellum: case report. Turkiye Klin. Tip. Bilimleri Derg. 32, 1724–1728 (2012).
Google Scholar
Trillet, M., Vighetto, A., Croisile, B., Charles, N. & Aimard, G. Hemiballismus with logorrhea and thymo-affective disinhibition caused by hematoma of the left subthalamic nucleus. Rev. Neurologique. 151, 416–419 (1995).
CAS
Google Scholar
Trimble, M. R. & Cummings, J. L. Neuropsychiatric disturbances following brainstem lesions. Br. J. Psychiatry 138, 56–59 (1981).
CAS
PubMed
Google Scholar
Turecki, G., Mari Jde, J. & Del Porto, J. A. Bipolar disorder following a left basal-ganglia stroke. Br. J. Psychiatry.: J. Ment. Sci. 163, 690 (1993).
CAS
Google Scholar
Vidrih, B., Karlovic, D. & Pasic, M. B. Arachnoid cyst as the cause of bipolar affective disorder: case report. Acta Clin. Croatica. 51, 655–659 (2012).
Google Scholar
Wright, M. T., Cummings, J. L., Mendez, M. F. & Foti, D. J. Bipolar syndromes following brain trauma. Neurocase 3, 111–118 (1997).
Google Scholar
Ybarra, M. I., Moreira, M. A., Araujo, C. R., Lana-Peixoto, M. A. & Lucio Teixeira, A. Bipolar disorder and multiple sclerosis. Arquivos Neuro Psiquiatr. 65(4B), 1177–1180 (2007).
Google Scholar
Yetimalar, Y., Iyidogan, E. & Basoglu, M. Secondary mania after pontin cavernous angioma. J. Neuropsychiatry Clin. Neurosci. 19, 344–345 (2007).
PubMed
Google Scholar
Zincir, S. B., Izci, F. & Acar, G. Mania secondary to traumatic brain injury: a case report. J. neuropsychiatry Clin. Neurosci. 26, E31 (2014).
PubMed
Google Scholar
Rush, B. in Encyclopedia of Clinical Neuropsychology 1065–1065 (Springer, 2011).
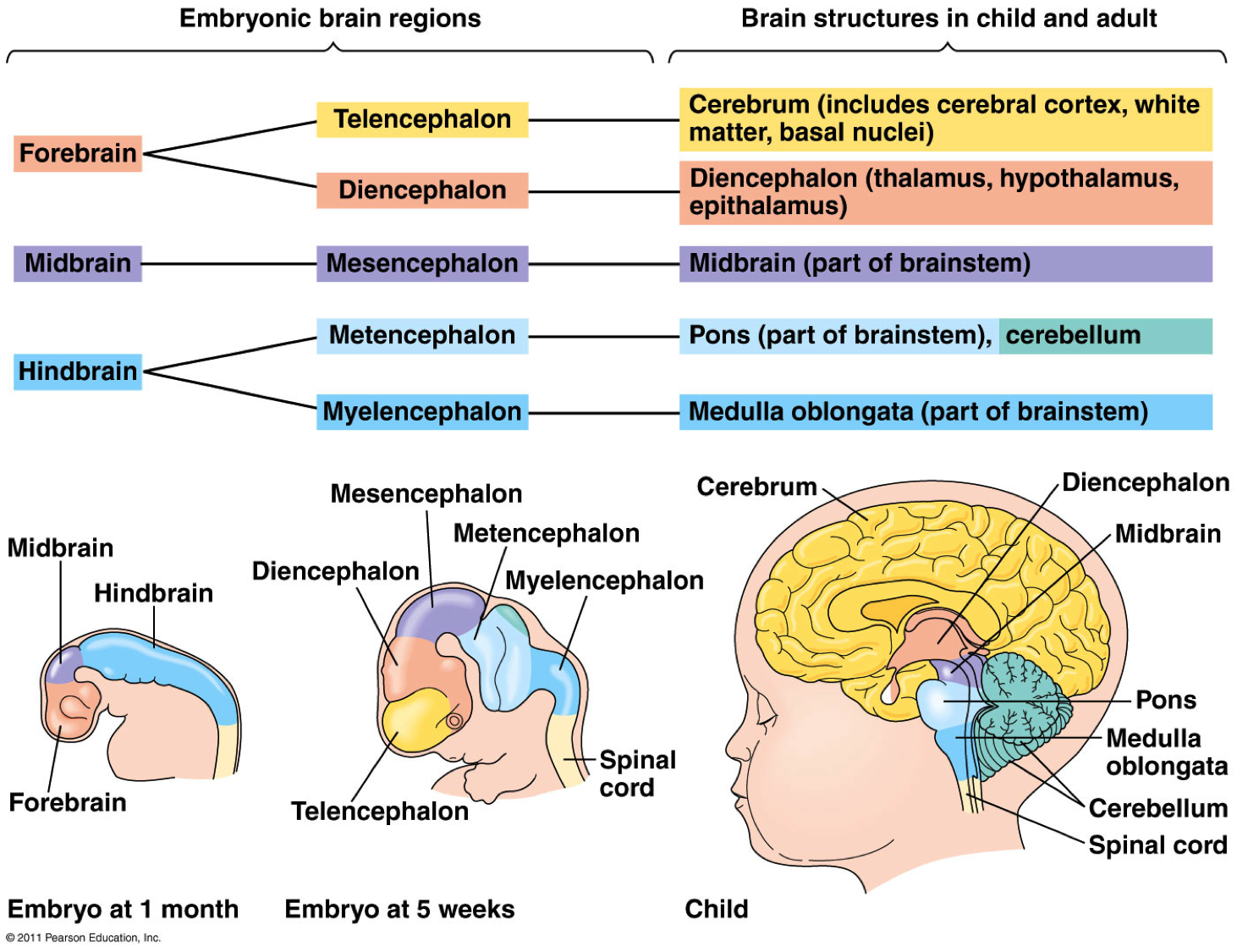
Selvaraj, S. et al. Grey matter differences in bipolar disorder: a meta‐analysis of voxel‐based morphometry studies. Bipolar Disord. 14, 135–145 (2012).
PubMed
Google Scholar
Wise, T. et al. Voxel-based meta-analytical evidence of structural disconnectivity in major depression and bipolar disorder. Biol. Psychiatry 79, 293–302 (2016).
PubMed
Google Scholar
Wise, T. et al. Common and distinct patterns of grey-matter volume alteration in major depression and bipolar disorder: evidence from voxel-based meta-analysis. Mol. Psychiatry 22, 1455 (2017).
CAS
PubMed
Google Scholar
Wang, Y. et al. Disrupted resting-state functional connectivity in nonmedicated bipolar disorder. Radiology 000, 151641–151641 (2016).
Google Scholar
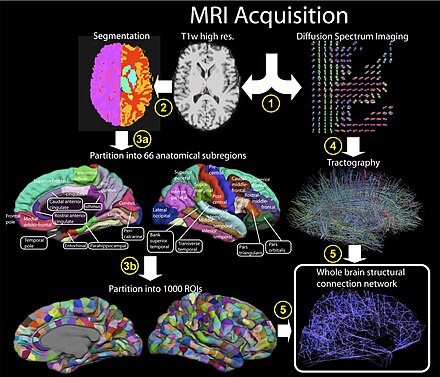
Strakowski, S. M. et al. fMRI brain activation in bipolar mania: Evidence for disruption of the ventrolateral prefrontal-amygdala emotional pathway. Biol. Psychiatry 69, 381–388 (2011).
PubMed
Google Scholar
Blond, B. N., Fredericks, C. A. & Blumberg, H. P. Functional neuroanatomy of bipolar disorder: structure, function, and connectivity in an amygdala-anterior paralimbic neural system. Bipolar Disord. 14, 340–355 (2012).
PubMed
Google Scholar
Nusslock, R. et al. Waiting to win: Elevated striatal and orbitofrontal cortical activity during reward anticipation in euthymic bipolar disorder adults. Bipolar Disord. 14, 249–260 (2012).
PubMed
Google Scholar
Cao, B. et al. Hippocampal subfield volumes in mood disorders. Mol. Psychiatry 22, 1352–1358 (2017).
CAS
PubMed
PubMed Central
Google Scholar
Bora, E., Fornito, A., Yücel, M. & Pantelis, C. Voxelwise meta-analysis of gray matter abnormalities in bipolar disorder. Biol. Psychiatry 67, 1097–1105 (2010).
PubMed
Google Scholar
Clark, L. & Sahakian, B. J. Cognitive neuroscience and brain imaging in bipolar disorder. Int. J. Psychophysiol. 10, 153–163 (2008).
Google Scholar
Hibar, D. P. et al. Subcortical volumetric abnormalities in bipolar disorder. Mol. Psychiatry 21, 1710 (2016).
CAS
PubMed
PubMed Central
Google Scholar
Hibar, D. P. et al. Cortical abnormalities in bipolar disorder: an MRI analysis of 6503 individuals from the ENIGMA Bipolar Disorder Working Group. Mol. Psychiatry 23, 932 (2018).
CAS
Google Scholar
Perlman, S. B. et al. Emotional Face Processing in Pediatric Bipolar Disorder: Evidence for Functional Impairments in the Fusiform Gyrus. J. Am. Acad. Child Adolesc. Psychiatry 52, 1–19 (2013).
Google Scholar
Adleman, N. E. & Kayser, R. R. Abnormal fusiform activation during emotional-face encoding in children and adults with bipolar disorder. Psychiatry Res. Neuroimaging 212, 161–163 (2013).
Google Scholar
Yasuno, F. et al. Interhemispheric functional disconnection because of abnormal corpus callosum integrity in bipolar disorder type II. Br. J. Psychiatry Open 2, 335–340 (2016).
Google Scholar
Karnath, H.-O., Fruhmann Berger, M. , Küker, W. & Rorden, C. The anatomy of spatial neglect based on voxelwise statistical analysis: a study of 140 patients. Cereb. Cortex 14, 1164–1172 (2004).
PubMed
Google Scholar
Buckner, R. L., Andrews-Hanna, J. R. & Schacter, D. L. The brain’s default network: Anatomy, function, and relevance to disease. Ann. NY Acad. Sci. 1124, 1–38 (2008).
PubMed
Google Scholar
Haldane, M., Cunningham, G., Androutsos, C. & Frangou, S. Structural brain correlates of response inhibition in Bipolar Disorder I. J. Psychopharmacol. 22, 138–143 (2008).
PubMed
Google Scholar
Tost, H. et al. Prefrontal-temporal gray matter deficits in bipolar disorder patients with persecutory delusions. J. Affect. Disord. 120, 54–61 (2010).
PubMed
Google Scholar

Liu, C.-H. et al. Regional homogeneity within the default mode network in bipolar depression: a resting-state functional magnetic resonance imaging study. PLoS One. 7, e48181–e48181 (2012).
CAS
PubMed
PubMed Central
Google Scholar
Dosenbach, N. U. et al. Distinct brain networks for adaptive and stable task control in humans. Proc. Natl Acad. Sci. 104, 11073–11078 (2007).
CAS
PubMed
Google Scholar
Kam, J. W. et al. Default network and frontoparietal control network theta connectivity supports internal attention. Nat. Hum. Behav. 3, 1263–1270 (2019).
PubMed
Google Scholar
Boes, A. D. et al. Network localization of neurological symptoms from focal brain lesions. Brain 138, 3061–3075 (2015).
PubMed
PubMed Central
Google Scholar
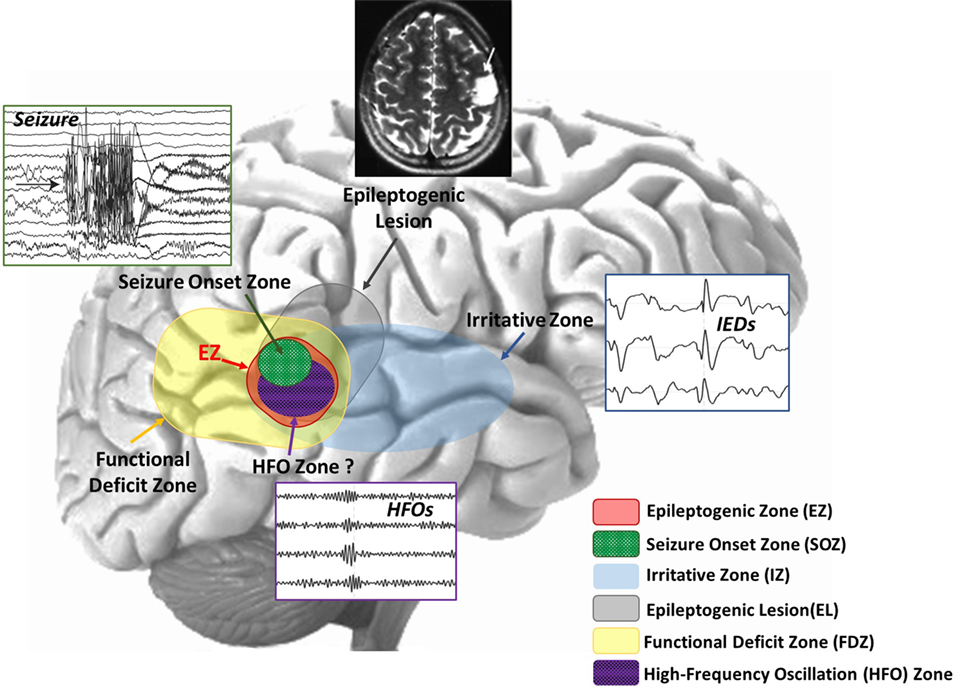
Darby, R. R., Laganiere, S., Pascual-Leone, A., Prasad, S. & Fox, M. D. Finding the imposter: brain connectivity of lesions causing delusional misidentifications. Brain 140, 497–507 (2016).
PubMed Central
Google Scholar
Yourganov, G., Smith, K. G., Fridriksson, J. & Rorden, C. Predicting aphasia type from brain damage measured with structural MRI. Cortex 73, 203–215 (2015).
PubMed
PubMed Central
Google Scholar
Darby, R. R., Horn, A., Cushman, F. & Fox, M. D. Lesion network localization of criminal behavior. Proc. Natl Acad. Sci. USA 115, 601–606 (2018).
CAS
PubMed
Google Scholar
Carson, A. J. et al. Depression after stroke and lesion location: a systematic review. Lancet 356, 122–126 (2000).
CAS
PubMed
Google Scholar

Vaidya, A. R., Pujara, M. S., Petrides, M., Murray, E. A. & Fellows, L. K. Lesion studies in contemporary neuroscience. Trends Cogn. Sci. 23, 653–671 (2019).
PubMed
PubMed Central
Google Scholar
Structural brain lesions in inflammatory bowel disease
World J Gastrointest Pathophysiol. 2015 Nov 15; 6(4): 124–130.
Can Dolapcioglu, Department of Gastroenterology, Dr. Lutfi Kirdar Kartal Research and Training Hospital, 34890 Istanbul, Turkey
Hatice Dolapcioglu, Department of Pathology, Fatih Sultan Mehmet Research and Training Hospital, 34752 Istanbul, Turkey
Author contributions: Both authors contributed to the conception and design of this work, to the acquisition and interpretation of the data, and to the drafting/writing of the manuscript.
Correspondence to: Dr. Can Dolapcioglu, Department of Gastroenterology, Dr. Lutfi Kirdar Kartal Research and Training Hospital, Şemsi Denizer Cad. E-5 Karayolu Cevizli Mevkii Kartal, 34890 Istanbul, Turkey. moc.liamtoh@icpalodnac
Telephone: +90-532-2613919 Fax: +90-216-4188752
Received 2015 Apr 20; Revised 2015 Jul 7; Accepted 2015 Aug 30.
Copyright ©The Author(s) 2015. Published by Baishideng Publishing Group Inc. All rights reserved.This article has been cited by other articles in PMC.
Abstract
Central nervous system (CNS) complications or manifestations of inflammatory bowel disease deserve particular attention because symptomatic conditions can require early diagnosis and treatment, whereas unexplained manifestations might be linked with pathogenic mechanisms. This review focuses on both symptomatic and asymptomatic brain lesions detectable on imaging studies, as well as their frequency and potential mechanisms. A direct causal relationship between inflammatory bowel disease (IBD) and asymptomatic structural brain changes has not been demonstrated, but several possible explanations, including vasculitis, thromboembolism and malnutrition, have been proposed. IBD is associated with a tendency for thromboembolisms; therefore, cerebrovascular thromboembolism represents the most frequent and grave CNS complication. Vasculitis, demyelinating conditions and CNS infections are among the other CNS manifestations of the disease. Biological agents also represent a risk factor, particularly for demyelination. Identification of the nature and potential mechanisms of brain lesions detectable on imaging studies would shed further light on the disease process and could improve patient care through early diagnosis and treatment.
Keywords: Inflammatory bowel disease, Ulcerative colitis, Crohn’s disease, Structural lesions, Magnetic resonance imaging, Brain lesions
Core tip: Central nervous system complications or manifestations of inflammatory bowel disease deserve particular attention because symptomatic conditions can require early diagnosis and treatment, whereas unexplained manifestations might be linked to pathogenic mechanisms. This review focuses on both symptomatic and asymptomatic brain lesions detectable on imaging studies, as well as their frequency and potential mechanisms. A direct causal relationship between inflammatory bowel disease and asymptomatic structural brain changes has not been demonstrated, but several possible explanations, including vasculitis, thromboembolism and malnutrition, have been proposed. Identification of the nature and potential mechanisms of brain lesions on imaging studies would improve patient care through early diagnosis and treatment.
INTRODUCTION
Inflammatory bowel diseases (IBDs), namely ulcerative colitis (UC) and Crohn’s disease (CD), are chronic, debilitating conditions with their onset at relatively young ages. CD is a transmural disease of gastrointestinal mucosa, and it has the potential to affect the entire gastrointestinal tract; in contrast, UC is not a transmural disease, and it affects the colon[1,2]. Both have relapsing and remitting courses. It is estimated that the total (UC plus CD) prevalence of IBD is approximately 0. 4% in the Western populations[3].
Because IBD can involve body parts other than the gastrointestinal tract, it can be regarded as a systemic disease. Involvement of the skin, eyes, joints, liver, biliary tract, kidneys, and bone, as well as hematological, and neurological involvement, can occur, preceding, accompanying or following gastrointestinal symptoms. These extraintestinal manifestations are more common in CD patients, although a substantial number of IBD patients can develop these conditions[4-6].
Neurological manifestations are relatively rare but are of clinical importance, particularly in terms of the need for timely diagnosis and management. Several mechanisms, including thromboembolisms, immunologic abnormalities, drug side effects, malabsorption, and infections, have been suggested as pathogeneses[7]. Most central neurological manifestations of IBD can be detected by brain imaging studies because they cause structural alterations of neural structures to some extent. However, lesions of unknown clinical relevance have also been detected in these patients, with significantly higher prevalence than in the normal population.
This review focuses in particular on structural brain lesions with positive findings on imaging studies, including symptomatic conditions and asymptomatic situations with brain lesions as well (Table ).
Table 1
Asymptomatic structural changes |
Symptomatic structural changes |
Cerebrovascular lesions |
Demyelinating conditions |
Cerebral vasculitis |
CNS infections |
ASYMPTOMATIC STRUCTURAL CHANGES ON MAGNETIC RESONANCE IMAGING
To date, a small number of studies have examined the presence of white matter lesions and other structural alterations on imaging studies in patients with IBD. These lesions were asymptomatic with potential associations with IBD, and whether these structural changes represent a unique extraintestinal manifestation of the disease remains unclear. Figure depicts white matter and gray matter (GM) on an magnetic resonance imaging (MRI) scan.
T1-weighted magnetic resonance image of a coronal section through the brain. Gray matter and white matter are indicated, as well as the ventricles.
Initial reports examining the associations between IBD and asymptomatic brain lesions were conflicting[8,9]. In a study by Geissler et al[8], 72 patients with IBD (48 cases of CD and 24 of UC) and 50 healthy age-matched controls underwent magnetic resonance imaging with gadolinium-enhanced studies. In that series, hyperintense focal white-matter lesions of 2-8 mm in diameter were found in 42% and 46% of patients with CD and UC, respectively, whereas such lesions were only present in 16% of healthy controls, resulting in relative risks of 2.6 for CD (95%CI: 1. 3-5.3) and 2.9 for UC (95%CI: 1.3-6.2). A longer duration of disease and older age were associated with an increased tendency for the lesions, and none of the patients had neurological symptoms. In contrast to the study by Geissler et al[8], Hart et al[9] did not find a significantly increased frequency of asymptomatic brain white matter lesions on the MRIs of IBD patients (n = 40), compared to a control group consisting of 40 age- and sex-matched patients admitted for tension-type headache (12.5% vs 5%, P = 0.43). Although the relatively small sample size of the latter study might have prevented the differences from attaining statistical significance, the authors emphasized that such asymptomatic lesions had previously been reported consistently in healthy subjects[10], and they expressed concerns about the clinical relevance of these findings for patients with IBD. However, it should be emphasized that both reports dated from two decades ago. In contrast, a recent study with a relatively small sample size also could not find an increased rate of white matter lesions among patients with IBD, compared to healthy controls[11].
In that study, the frequencies of white matter lesions and other brainstem parenchymal lesions were similar, but among the subjects with white matter lesions, the number of lesions was significantly higher in IBD patients.
Two recent studies compared the frequency of white matter lesions between IBD patients and normal subjects using advanced magnetic resonance imaging techniques and equipment[12,13]. Chen et al[12] found a very high prevalence of hyperintense white matter lesions in patients with CD, compared to age-matched controls (75% vs 34%, P < 0.001). Their study had a relatively large sample size (54 Crohn’s patients and 100 age-matched controls). Similarly, Zikou et al[13] found a significantly increased frequency of white matter lesions among patients with IBD (Crohn’s and UC), compared to controls (66% vs 45%, P < 0.05). Both studies used fluid-attenuated inversion recovery images to evaluate white matter hyperintensities.
The development of advanced MRI techniques has allowed for better examination of brain structures, including estimation of volume differences and the evaluation of microstructural integrity. Voxel-based morphometry is a technique used to compare the regional brain volumes of subjects, and it uses MRI data. Several studies using this technique have found a decreased GM volume among IBD patients, compared to controls. Agostini et al[14], in a study of CD patients, found decreased GM volume in parts of the frontal gyrus and in the anterior midcingulate cortex. In addition, negative correlations between disease duration and GM volume in several brain regions were found in the former study. In contrast, a recent study by the same lead author did not identify any decrease in GM volume in UC patients, compared to controls[15]. Zikou et al[13], however, found more diffuse GM volume decreases in a sample of IBD patients, consisting of subjects with CD and UC, involving the right and left fusiform gyri, the right and left temporal inferior gyri, the right precentral gyrus, the right superior motor area, the right middle frontal gyrus and the left superior parietal gyrus.
Diffusion tensor imaging (DTI) is an MRI technique that measures molecular diffusion. It is valuable for the identification of axonal injury and can be useful in differentiating between primary and Wallerian degeneration[16]. To date, only a single study has examined the brains of IBD patients using DTI techniques and compared them with age-matched healthy controls[13]. That study found decreased white matter axial diffusivity in the right corticospinal tract and the right superior longitudinal fasciculus in IBD patients (Crohn’s and UC) compared to controls, indicating a possible degree of change in neural structures[17-21].
Several possible explanations have been proposed for the increased prevalence of white matter lesions, the decreased GM volume, and the decreased diffusivity in major tracks among patients with IBD. White matter lesions on brain MRI examinations have been associated with many conditions, including migraine headaches, hypertension, diabetes, celiac disease, and cerebrovascular disease, as well as being found in healthy subjects[10,22-26]. The increased frequency of white matter hyperintensities in IBD patients might be due to central nervous system (CNS) vasculitis, likely secondary to coagulation and vessel obstruction[13]. IBD is known to be associated with thromboembolism and hypercoagulability[27-29]. Another possible explanation is malabsorption because such lesions were previously described in celiac disease[26].
One explanation for decreased GM volume might be excito-toxicity due to chronic pain, which can result in neural atrophy or loss[14,30]. Structural changes of varying types have been observed in pain-related brain regions in other chronic pain syndromes[31-36], with GM decreases in the frontal and cingulate cortices being the most common anomalies[30]. Another possible mechanism might be increased inflammatory cytokines, resulting in astrocyte and oligodendrocyte apoptosis, decreased neurogenesis, and increased oxidative stress, thus leading to GM volume loss[37,38]. Similarly, cerebral small vessel vasculitis and the neurotoxic effects of cytokines could be responsible for the decreased axial diffusivity in major tracts[13].
Although a direct causal relationship between IBD and asymptomatic structural brain changes cannot be established, the available findings and their implications deserve further investigation, particularly in the context of the brain functional changes observed in patients with IBD[39].
CEREBROVASCULAR LESIONS
Patients with IBD have an increased tendency for thrombotic events so that IBD could be considered a prothrombotic condition that increases the risk of cerebral arterial and venous thrombosis[40-44]. A recent meta-analysis found a modestly increased risk of cerebrovascular accidents among patients with IBD (OR = 1.18)[45]. The increased risk was more prominent among female patients and young patients. Venous thrombosis has also been seen in these patients with remarkable frequency[44,46-49]. Cerebrovascular events have been documented in up to 4% of IBD patients[50]. These cases are of particular clinical importance when the consequences of these conditions and the young age of the patient population are considered.
A number of pathological conditions have been linked to IBD in attempts to propose a mechanism for the increased tendency for thromboembolic events, including abnormalities of platelets and coagulation factors, genetic mutations, vitamin B6 deficiency due to hypercatabolism and malabsorption, antiphospholipid antibodies, hyperhomocystinemia, dehydration, and immobilization[4,7,40,49,51-58]. However, to date, the exact mechanism has not been fully understood.
Cerebral arterial thromboembolism can present with headache, paresis, seizures or dysphagia, and it can result in high mortality and morbidity[51]. Large infarcts involving both the anterior and posterior circulation and lacunar infarcts have been reported in IBD[59-63]. In addition, UC has been associated with thrombotic thrombocytopenic purpura and small and large cerebral artery thrombosis risks[64-66]. Infarcts associated with IBD can be identified on computerized tomography and magnetic resonance imaging.
Cerebral venous thrombosis and sinus thrombosis seem to be more frequent in patients with UC than in CD patients[67]. Most often, the superior sagittal sinus and lateral sinuses are involved; however, thrombosis of the cortical venous sinuses has also been reported[68]. Young and male patients seem to be at greater risk[50,69,70]. The most common presenting symptom is headache, usually followed by neurological impairment, and cerebral infarction can develop due to extension of thrombus[71]. A combination of magnetic resonance imaging and magnetic resonance venography could identify venous occlusion[71]. The radiological characteristics, as well as the clinical course and prognosis of IBD-related cerebral venous thrombosis, seem to be similar to those in cases not related to IBD[44,72,73].
DEMYELINATING CONDITIONS
Demyelinating conditions have been reported in the setting of IBD, in association or not with biological treatments.
Multiple sclerosis (MS) or MS-like conditions have long been reported in patients with IBD[74-77]. Such a relationship was first reported by Rang et al[74]. A retrospective study found an increased incidence of demyelinating disease among patients with IBD, particularly in UC[77]. Nevertheless, MS has been reported in both UC and CD[78,79]. The findings of a recent meta-analysis supported a relationship between MS and IBD[80]. Both the development of an MS-like syndrome in the setting of IBD and the development of IBD in MS patients have been reported[81-83]. Because the diagnostic criteria for MS have evolved over time, some lesions found in previous studies might not actually be MS; rather, they could represent an MS-like syndrome, which might potentially be linked to IBD. The mechanism of these relationships has not been fully explained, but a role for impairments in functional T-cell subsets has been proposed[4,57].
Anti-tumor necrosis factor (TNF)-alpha drugs and anti-alpha4 integrin drugs (such as natalizumab), which are biological agents used in the treatment of IBD, can have adverse neurological effects[84,85]. Progressive multifocal leukoencephalopathy is the gravest complication, particularly when associated with natalizumab therapy, although few cases have been reported in association with TNF-alpha drugs[85]. Reactivation of John Cunningham virus (JCV) is responsible for the development of PML, and it is associated with visual defects, mental impairment, confusion and personality changes, followed by motor weakness[57]. MRI is helpful in the diagnosis, showing white matter lesions with typical T1 and T2 signals[86]. Diagnosis can be confirmed by polymerase chain reaction for JCV DNA. Despite treatment, PML has a high mortality rate of 60%[87]. In addition, development/exacerbation of MS or demyelination has been reported in association with anti-TNF-alpha therapy[88-90].
CEREBRAL VASCULITIS
Cerebral vasculitis has been reported in patients with UC in a number of studies[91-96]. In addition, a case of cerebral vasculitis was reported in association with CD[97]. Mostly immune-mediated mechanisms have been proposed for the development of vasculitis in UC. Magnetic resonance imaging is abnormal and shows hyperintense lesions[95,96], and magnetic resonance angiography can aid in diagnosis[97]. CNS vasculitis has also been reported in association with anti-TNF therapy[57]. The major symptoms of cerebral vasculitis are stroke, headache and encephalopathy. Other symptoms include seizures, cranial nerve palsies or myelopathies[98]. Cerebral vasculitis mimicking migraine with aura was reported in a case of CD, and the authors stated that migraine with aura can be the only finding in cerebral vasculitis[99]. Cerebral vasculitis resulting in stroke has been rarely reported in UC[61]. Cerebral vasculitis presented with right paresis and unbalanced gait in a 35-year-old woman with UC[93]. Another UC case was complicated by convulsions and was diagnosed as cerebral vasculitis on magnetic resonance imaging[96].
CNS INFECTIONS
Anti-TNF agents can suppress the immune system to such an extent that opportunistic infections develop, including of the CNS, in IBD patients. These patients present with meningeal signs, seizures, symptoms resembling stroke, and encephalopathy[100]; abnormal MRI findings and/or mass lesions are found on imaging studies. Among these opportunistic infections are fungal infections, cerebral tuberculosis, Epstein-Barr virus infection, nocardiosis, toxoplasmosis, herpes simplex virus infection, meningococcal infection, Campylobacter fetus infections, and listeria infections[57]. In a severe case of CD with ileocecal involvement, opportunistic meningitis with varicella zoster was reported after adalimumab and prednisone treatment[101]. In a patient with CD, meningococcal meningoencephalitis was reported after certolizumab pegol treatment[102].
Footnotes
P- Reviewer: Lakatos PL, Swaminath A S- Editor: Ma YJ L- Editor: A E- Editor: Liu SQ
Conflict-of-interest statement: The authors do not report any conflict of interest regarding this manuscript.
Open-Access: This article is an open-access article which was selected by an in-house editor and fully peer-reviewed by external reviewers. It is distributed in accordance with the Creative Commons Attribution Non Commercial (CC BY-NC 4.0) license, which permits others to distribute, remix, adapt, build upon this work non-commercially, and license their derivative works on different terms, provided the original work is properly cited and the use is non-commercial. See: http://creativecommons.org/licenses/by-nc/4.0/
Peer-review started: April 21, 2015
First decision: May 18, 2015
Article in press: September 7, 2015
References
1. Baumgart DC, Sandborn WJ. Inflammatory bowel disease: clinical aspects and established and evolving therapies. Lancet. 2007;369:1641–1657. [PubMed] [Google Scholar]2. Baumgart DC, Carding SR. Inflammatory bowel disease: cause and immunobiology. Lancet. 2007;369:1627–1640. [PubMed] [Google Scholar]4. Morís G. Inflammatory bowel disease: an increased risk factor for neurologic complications. World J Gastroenterol. 2014;20:1228–1237. [PMC free article] [PubMed] [Google Scholar]5. Veloso FT. Extraintestinal manifestations of inflammatory bowel disease: do they influence treatment and outcome? World J Gastroenterol. 2011;17:2702–2707. [PMC free article] [PubMed] [Google Scholar]6. Bernstein CN, Blanchard JF, Rawsthorne P, Yu N. The prevalence of extraintestinal diseases in inflammatory bowel disease: a population-based study. Am J Gastroenterol. 2001;96:1116–1122. [PubMed] [Google Scholar]7. Zois CD, Katsanos KH, Kosmidou M, Tsianos EV. Neurologic manifestations in inflammatory bowel diseases: current knowledge and novel insights.
J Crohns Colitis. 2010;4:115–124. [PubMed] [Google Scholar]8. Geissler A, Andus T, Roth M, Kullmann F, Caesar I, Held P, Gross V, Feuerbach S, Schölmerich J. Focal white-matter lesions in brain of patients with inflammatory bowel disease. Lancet. 1995;345:897–898. [PubMed] [Google Scholar]9. Hart PE, Gould SR, MacSweeney JE, Clifton A, Schon F. Brain white-matter lesions in inflammatory bowel disease. Lancet. 1998;351:1558. [PubMed] [Google Scholar]10. Horikoshi T, Yagi S, Fukamachi A. Incidental high-intensity foci in white matter on T2-weighted magnetic resonance imaging. Frequency and clinical significance in symptom-free adults. Neuroradiology. 1993;35:151–155. [PubMed] [Google Scholar]11. Dolapcioglu C, Guleryuzlu Y, Uygur-Bayramicli O, Ahishali E, Dabak R. Asymptomatic brain lesions on cranial magnetic resonance imaging in inflammatory bowel disease. Gut Liver. 2013;7:169–174. [PMC free article] [PubMed] [Google Scholar]12. Chen M, Lee G, Kwong LN, Lamont S, Chaves C. Cerebral white matter lesions in patients with Crohn’s disease.
J Neuroimaging. 2012;22:38–41. [PubMed] [Google Scholar]13. Zikou AK, Kosmidou M, Astrakas LG, Tzarouchi LC, Tsianos E, Argyropoulou MI. Brain involvement in patients with inflammatory bowel disease: a voxel-based morphometry and diffusion tensor imaging study. Eur Radiol. 2014;24:2499–2506. [PubMed] [Google Scholar]14. Agostini A, Benuzzi F, Filippini N, Bertani A, Scarcelli A, Farinelli V, Marchetta C, Calabrese C, Rizzello F, Gionchetti P, et al. New insights into the brain involvement in patients with Crohn’s disease: a voxel-based morphometry study. Neurogastroenterol Motil. 2013;25:147–e82. [PubMed] [Google Scholar]15. Agostini A, Campieri M, Bertani A, Scarcelli A, Ballotta D, Calabrese C, Rizzello F, Gionchetti P, Nichelli P, Benuzzi F. Absence of change in the gray matter volume of patients with ulcerative colitis in remission: a voxel based morphometry study. Biopsychosoc Med. 2015;9:1. [PMC free article] [PubMed] [Google Scholar]16. Pierpaoli C, Barnett A, Pajevic S, Chen R, Penix LR, Virta A, Basser P.
Water diffusion changes in Wallerian degeneration and their dependence on white matter architecture. Neuroimage. 2001;13:1174–1185. [PubMed] [Google Scholar]17. Beaulieu C. The basis of anisotropic water diffusion in the nervous system – a technical review. NMR Biomed. 2002;15:435–455. [PubMed] [Google Scholar]18. Zivadinov R, Shucard JL, Hussein S, Durfee J, Cox JL, Bergsland N, Dwyer MG, Benedict RH, Ambrus J, Shucard DW. Multimodal imaging in systemic lupus erythematosus patients with diffuse neuropsychiatric involvement. Lupus. 2013;22:675–683. [PubMed] [Google Scholar]19. Pievani M, Paternicò D, Benussi L, Binetti G, Orlandini A, Cobelli M, Magnaldi S, Ghidoni R, Frisoni GB. Pattern of structural and functional brain abnormalities in asymptomatic granulin mutation carriers. Alzheimers Dement. 2014;10:S354–S363.e1. [PubMed] [Google Scholar]20. Padilla N, Junqué C, Figueras F, Sanz-Cortes M, Bargalló N, Arranz A, Donaire A, Figueras J, Gratacos E. Differential vulnerability of gray matter and white matter to intrauterine growth restriction in preterm infants at 12 months corrected age.
Brain Res. 2014;1545:1–11. [PubMed] [Google Scholar]21. Adamson C, Yuan W, Babcock L, Leach JL, Seal ML, Holland SK, Wade SL. Diffusion tensor imaging detects white matter abnormalities and associated cognitive deficits in chronic adolescent TBI. Brain Inj. 2013;27:454–463. [PMC free article] [PubMed] [Google Scholar]22. Bryan RN, Manolio TA, Schertz LD, Jungreis C, Poirier VC, Elster AD, Kronmal RA. A method for using MR to evaluate the effects of cardiovascular disease on the brain: the cardiovascular health study. AJNR Am J Neuroradiol. 1994;15:1625–1633. [PMC free article] [PubMed] [Google Scholar]23. Meguro K, Yamaguchi T, Hishinuma T, Miyazawa H, Ono S, Yamada K, Matsuzawa T. Periventricular hyperintensity on magnetic resonance imaging correlated with brain ageing and atrophy. Neuroradiology. 1993;35:125–129. [PubMed] [Google Scholar]24. Swartz RH, Kern RZ. Migraine is associated with magnetic resonance imaging white matter abnormalities: a meta-analysis. Arch Neurol. 2004;61:1366–1368.
[PubMed] [Google Scholar]25. Pavese N, Canapicchi R, Nuti A, Bibbiani F, Lucetti C, Collavoli P, Bonuccelli U. White matter MRI hyperintensities in a hundred and twenty-nine consecutive migraine patients. Cephalalgia. 1994;14:342–345. [PubMed] [Google Scholar]26. Kieslich M, Errázuriz G, Posselt HG, Moeller-Hartmann W, Zanella F, Boehles H. Brain white-matter lesions in celiac disease: a prospective study of 75 diet-treated patients. Pediatrics. 2001;108:E21. [PubMed] [Google Scholar]27. Novacek G, Miehsler W, Kapiotis S, Katzenschlager R, Speiser W, Vogelsang H. Thromboembolism and resistance to activated protein C in patients with inflammatory bowel disease. Am J Gastroenterol. 1999;94:685–690. [PubMed] [Google Scholar]28. Lam A, Borda IT, Inwood MJ, Thomson S. Coagulation studies in ulcerative colitis and Crohn’s disease. Gastroenterology. 1975;68:245–251. [PubMed] [Google Scholar]29. Twig G, Zandman-Goddard G, Szyper-Kravitz M, Shoenfeld Y. Systemic thromboembolism in inflammatory bowel disease: mechanisms and clinical applications.
Ann N Y Acad Sci. 2005;1051:166–173. [PubMed] [Google Scholar]30. May A. Chronic pain may change the structure of the brain. Pain. 2008;137:7–15. [PubMed] [Google Scholar]31. Kuchinad A, Schweinhardt P, Seminowicz DA, Wood PB, Chizh BA, Bushnell MC. Accelerated brain gray matter loss in fibromyalgia patients: premature aging of the brain? J Neurosci. 2007;27:4004–4007. [PMC free article] [PubMed] [Google Scholar]32. Apkarian AV, Sosa Y, Sonty S, Levy RM, Harden RN, Parrish TB, Gitelman DR. Chronic back pain is associated with decreased prefrontal and thalamic gray matter density. J Neurosci. 2004;24:10410–10415. [PMC free article] [PubMed] [Google Scholar]33. Schmidt-Wilcke T, Leinisch E, Straube A, Kämpfe N, Draganski B, Diener HC, Bogdahn U, May A. Gray matter decrease in patients with chronic tension type headache. Neurology. 2005;65:1483–1486. [PubMed] [Google Scholar]34. Blankstein U, Chen J, Diamant NE, Davis KD. Altered brain structure in irritable bowel syndrome: potential contributions of pre-existing and disease-driven factors.
Gastroenterology. 2010;138:1783–1789. [PubMed] [Google Scholar]35. Seminowicz DA, Labus JS, Bueller JA, Tillisch K, Naliboff BD, Bushnell MC, Mayer EA. Regional gray matter density changes in brains of patients with irritable bowel syndrome. Gastroenterology. 2010;139:48–57.e2. [PMC free article] [PubMed] [Google Scholar]36. Schweinhardt P, Kuchinad A, Pukall CF, Bushnell MC. Increased gray matter density in young women with chronic vulvar pain. Pain. 2008;140:411–419. [PubMed] [Google Scholar]37. Miller AH, Maletic V, Raison CL. Inflammation and its discontents: the role of cytokines in the pathophysiology of major depression. Biol Psychiatry. 2009;65:732–741. [PMC free article] [PubMed] [Google Scholar]38. Buntinx M, Moreels M, Vandenabeele F, Lambrichts I, Raus J, Steels P, Stinissen P, Ameloot M. Cytokine-induced cell death in human oligodendroglial cell lines: I. Synergistic effects of IFN-gamma and TNF-alpha on apoptosis. J Neurosci Res. 2004;76:834–845. [PubMed] [Google Scholar]39.
Agostini A, Filippini N, Cevolani D, Agati R, Leoni C, Tambasco R, Calabrese C, Rizzello F, Gionchetti P, Ercolani M, et al. Brain functional changes in patients with ulcerative colitis: a functional magnetic resonance imaging study on emotional processing. Inflamm Bowel Dis. 2011;17:1769–1777. [PubMed] [Google Scholar]40. Canero A, Parmeggiani D, Avenia N, Atelli PF, Goffredi L, Peltrini R, Madonna I, Ambrosino P, Apperti S, Apperti M. Thromboembolic tendency (TE) in IBD (Inflammatory bowel disease) patients. Ann Ital Chir. 2012;83:313–317. [PubMed] [Google Scholar]41. Kappelman MD, Horvath-Puho E, Sandler RS, Rubin DT, Ullman TA, Pedersen L, Baron JA, Sørensen HT. Thromboembolic risk among Danish children and adults with inflammatory bowel diseases: a population-based nationwide study. Gut. 2011;60:937–943. [PubMed] [Google Scholar]42. Barclay AR, Keightley JM, Horrocks I, Garrick V, McGrogan P, Russell RK. Cerebral thromboembolic events in pediatric patients with inflammatory bowel disease.
Inflamm Bowel Dis. 2010;16:677–683. [PubMed] [Google Scholar]43. Benavente L, Morís G. Neurologic disorders associated with inflammatory bowel disease. Eur J Neurol. 2011;18:138–143. [PubMed] [Google Scholar]44. Cognat E, Crassard I, Denier C, Vahedi K, Bousser MG. Cerebral venous thrombosis in inflammatory bowel diseases: eight cases and literature review. Int J Stroke. 2011;6:487–492. [PubMed] [Google Scholar]45. Singh S, Singh H, Loftus EV, Pardi DS. Risk of cerebrovascular accidents and ischemic heart disease in patients with inflammatory bowel disease: a systematic review and meta-analysis. Clin Gastroenterol Hepatol. 2014;12:382–93.e1: quiz e22. [PubMed] [Google Scholar]46. Nudelman RJ, Rosen DG, Rouah E, Verstovsek G. Cerebral sinus thrombosis: a fatal neurological complication of ulcerative colitis. Patholog Res Int. 2010;2010:132754. [PMC free article] [PubMed] [Google Scholar]47. Casella G, Spreafico C, Costantini M, Pagni F, Cortelezzi CC, Baldini V, DeLodovici ML, Bono G. Cerebral sinus thrombosis in ulcerative colitis.
Inflamm Bowel Dis. 2011;17:2214–2216. [PubMed] [Google Scholar]48. Katsanos AH, Katsanos KH, Kosmidou M, Giannopoulos S, Kyritsis AP, Tsianos EV. Cerebral sinus venous thrombosis in inflammatory bowel diseases. QJM. 2013;106:401–413. [PubMed] [Google Scholar]49. Ferro JM, Oliveira S. Neurologic manifestations of gastrointestinal and liver diseases. Curr Neurol Neurosci Rep. 2014;14:487. [PubMed] [Google Scholar]50. Miehsler W, Reinisch W, Valic E, Osterode W, Tillinger W, Feichtenschlager T, Grisar J, Machold K, Scholz S, Vogelsang H, et al. Is inflammatory bowel disease an independent and disease specific risk factor for thromboembolism? Gut. 2004;53:542–548. [PMC free article] [PubMed] [Google Scholar]51. Saibeni S, Spina L, Vecchi M. Exploring the relationships between inflammatory response and coagulation cascade in inflammatory bowel disease. Eur Rev Med Pharmacol Sci. 2004;8:205–208. [PubMed] [Google Scholar]52. Dolapcioglu C, Soylu A, Kendir T, Ince AT, Dolapcioglu H, Purisa S, Bolukbas C, Sokmen HM, Dalay R, Ovunc O.
Coagulation parameters in inflammatory bowel disease. Int J Clin Exp Med. 2014;7:1442–1448. [PMC free article] [PubMed] [Google Scholar]53. Tsiolakidou G, Koutroubakis IE. Thrombosis and inflammatory bowel disease-the role of genetic risk factors. World J Gastroenterol. 2008;14:4440–4444. [PMC free article] [PubMed] [Google Scholar]54. Chamouard P, Grunebaum L, Wiesel ML, Freyssinet JM, Duclos B, Cazenave JP, Baumann R. Prevalence and significance of anticardiolipin antibodies in Crohn’s disease. Dig Dis Sci. 1994;39:1501–1504. [PubMed] [Google Scholar]55. Souto JC, Borrell M, Fontcuberta J, Roca M. Antiphospholipid antibodies in inflammatory bowel disease. Dig Dis Sci. 1995;40:1524–1525. [PubMed] [Google Scholar]56. Saibeni S, Cattaneo M, Vecchi M, Zighetti ML, Lecchi A, Lombardi R, Meucci G, Spina L, de Franchis R. Low vitamin B(6) plasma levels, a risk factor for thrombosis, in inflammatory bowel disease: role of inflammation and correlation with acute phase reactants. Am J Gastroenterol.
2003;98:112–117. [PubMed] [Google Scholar]57. Casella G, Tontini GE, Bassotti G, Pastorelli L, Villanacci V, Spina L, Baldini V, Vecchi M. Neurological disorders and inflammatory bowel diseases. World J Gastroenterol. 2014;20:8764–8782. [PMC free article] [PubMed] [Google Scholar]58. Danese S, Papa A, Saibeni S, Repici A, Malesci A, Vecchi M. Inflammation and coagulation in inflammatory bowel disease: The clot thickens. Am J Gastroenterol. 2007;102:174–186. [PubMed] [Google Scholar]59. Lossos A, Stener I. Cerebrovascular disease in inflammatory bowel disease. In: Bogousslavsky J, Kaplan L, editors. Uncommon causes of stroke. Cambridge: Cambridge University Press; 2001. pp. 161–168. [Google Scholar]60. Keene DL, Matzinger MA, Jacob PJ, Humphreys P. Cerebral vascular events associated with ulcerative colitis in children. Pediatr Neurol. 2001;24:238–243. [PubMed] [Google Scholar]61. Mayeux R, Fahn S. Strokes and ulcerative colitis. Neurology. 1978;28:571–574. [PubMed] [Google Scholar]62.
Schneiderman JH, Sharpe JA, Sutton DM. Cerebral and retinal vascular complications of inflammatory bowel disease. Ann Neurol. 1979;5:331–337. [PubMed] [Google Scholar]63. Fukuhara T, Tsuchida S, Kinugasa K, Ohmoto T. A case of pontine lacunar infarction with ulcerative colitis. Clin Neurol Neurosurg. 1993;95:159–162. [PubMed] [Google Scholar]64. Scheid R, Hegenbart U, Ballaschke O, Von Cramon DY. Major stroke in thrombotic-thrombocytopenic purpura (Moschcowitz syndrome) Cerebrovasc Dis. 2004;18:83–85. [PubMed] [Google Scholar]65. Baron BW, Jeon HR, Glunz C, Peterson A, Cohen R, Hanauer S, Rubin D, Hart J, Baron JM. First two patients with ulcerative colitis who developed classical thrombotic thrombocytopenic purpura successfully treated with medical therapy and plasma exchange. J Clin Apher. 2002;17:204–206. [PubMed] [Google Scholar]66. Hisada T, Miyamae Y, Mizuide M, Shibusawa N, Iida T, Masuo T, Okada S, Sagawa T, Ishizuka T, Kusano M, et al. Acute thrombocytopenia associated with preexisting ulcerative colitis successfully treated with colectomy.
Intern Med. 2006;45:87–91. [PubMed] [Google Scholar]67. Spina L, Saibeni S, Battaglioli T, Peyvandi F, de Franchis R, Vecchi M. Thrombosis in inflammatory bowel diseases: role of inherited thrombophilia. Am J Gastroenterol. 2005;100:2036–2041. [PubMed] [Google Scholar]68. Saadatnia M, Fatehi F, Basiri K, Mousavi SA, Mehr GK. Cerebral venous sinus thrombosis risk factors. Int J Stroke. 2009;4:111–123. [PubMed] [Google Scholar]69. Diakou M, Kostadima V, Giannopoulos S, Zikou AK, Argyropoulou MI, Kyritsis AP. Cerebral venous thrombosis in an adolescent with ulcerative colitis. Brain Dev. 2011;33:49–51. [PubMed] [Google Scholar]70. Benjilali L, Aidi S, El Mansouri H, Benabdejlil M, Jiddane M, El Alaoui Faris M. Cerebral thrombosis complicating Crohn’s disease: two cases. J Stroke Cerebrovasc Dis. 2011;20:565–569. [PubMed] [Google Scholar]71. Stam J. Thrombosis of the cerebral veins and sinuses. N Engl J Med. 2005;352:1791–1798. [PubMed] [Google Scholar]72. Ferro JM, Canhão P, Stam J, Bousser MG, Barinagarrementeria F.
Prognosis of cerebral vein and dural sinus thrombosis: results of the International Study on Cerebral Vein and Dural Sinus Thrombosis (ISCVT) Stroke. 2004;35:664–670. [PubMed] [Google Scholar]73. Rodríguez S, Calleja S, Morís G. Cluster-like headache heralding cerebral venous thrombosis. Cephalalgia. 2008;28:906–907. [PubMed] [Google Scholar]74. Rang EH, Brooke BN, Hermon-Taylor J. Association of ulcerative colitis with multiple sclerosis. Lancet. 1982;2:555. [PubMed] [Google Scholar]75. Pandian JD, Pawar G, Singh GS, Abraham R. Multiple sclerosis in a patient with chronic ulcerative colitis. Neurol India. 2004;52:282–283. [PubMed] [Google Scholar]76. Bernstein CN, Wajda A, Blanchard JF. The clustering of other chronic inflammatory diseases in inflammatory bowel disease: a population-based study. Gastroenterology. 2005;129:827–836. [PubMed] [Google Scholar]77. Gupta G, Gelfand JM, Lewis JD. Increased risk for demyelinating diseases in patients with inflammatory bowel disease. Gastroenterology.
2005;129:819–826. [PubMed] [Google Scholar]78. Scheid R, Teich N. Neurologic manifestations of ulcerative colitis. Eur J Neurol. 2007;14:483–493. [PubMed] [Google Scholar]79. Purrmann J, Arendt G, Cleveland S, Borchard F, Fürst W, Gemsa R, Bertrams J, Hengels KJ. Association of Crohn’s disease and multiple sclerosis. Is there a common background? J Clin Gastroenterol. 1992;14:43–46. [PubMed] [Google Scholar]80. Dobson R, Giovannoni G. Autoimmune disease in people with multiple sclerosis and their relatives: a systematic review and meta-analysis. J Neurol. 2013;260:1272–1285. [PubMed] [Google Scholar]81. Buccino GP, Corrente G, Visintini D. Crohn’s disease and multiple sclerosis: a single case report. Ital J Neurol Sci. 1994;15:303–306. [PMC free article] [PubMed] [Google Scholar]82. Kitchin LI, Knobler RL, Friedman LS. Crohn’s disease in a patient with multiple sclerosis. J Clin Gastroenterol. 1991;13:331–334. [PubMed] [Google Scholar]83. Beaugerie L, Lamy P, Ganne N, Carbonnel F, Le Quintrec Y, Cosnes J, Gendre JP.
[Morbid associations in Crohn’s disease. Study of a series of 832 patients] Presse Med. 1997;26:892–894. [PubMed] [Google Scholar]84. Deepak P, Stobaugh DJ, Ehrenpreis ED. Infectious complications of TNF-α inhibitor monotherapy versus combination therapy with immunomodulators in inflammatory bowel disease: analysis of the Food and Drug Administration Adverse Event Reporting System. J Gastrointestin Liver Dis. 2013;22:269–276. [PubMed] [Google Scholar]85. Van Assche G, Van Ranst M, Sciot R, Dubois B, Vermeire S, Noman M, Verbeeck J, Geboes K, Robberecht W, Rutgeerts P. Progressive multifocal leukoencephalopathy after natalizumab therapy for Crohn’s disease. N Engl J Med. 2005;353:362–368. [PubMed] [Google Scholar]86. Yousry TA, Major EO, Ryschkewitsch C, Fahle G, Fischer S, Hou J, Curfman B, Miszkiel K, Mueller-Lenke N, Sanchez E, et al. Evaluation of patients treated with natalizumab for progressive multifocal leukoencephalopathy. N Engl J Med. 2006;354:924–933. [PMC free article] [PubMed] [Google Scholar]87.
Singh S, Kumar N, Loftus EV, Kane SV. Neurologic complications in patients with inflammatory bowel disease: increasing relevance in the era of biologics. Inflamm Bowel Dis. 2013;19:864–872. [PubMed] [Google Scholar]88. Lozeron P, Denier C, Lacroix C, Adams D. Long-term course of demyelinating neuropathies occurring during tumor necrosis factor-alpha-blocker therapy. Arch Neurol. 2009;66:490–497. [PubMed] [Google Scholar]89. Pfueller CF, Seipelt E, Zipp F, Paul F. Multiple sclerosis following etanercept treatment for ankylosing spondylitis. Scand J Rheumatol. 2008;37:397–399. [PubMed] [Google Scholar]90. Lees CW, Ali AI, Thompson AI, Ho GT, Forsythe RO, Marquez L, Cochrane CJ, Aitken S, Fennell J, Rogers P, et al. The safety profile of anti-tumour necrosis factor therapy in inflammatory bowel disease in clinical practice: analysis of 620 patient-years follow-up. Aliment Pharmacol Ther. 2009;29:286–297. [PubMed] [Google Scholar]91. Nelson J, Barron MM, Riggs JE, Gutmann L, Schochet SS. Cerebral vasculitis and ulcerative colitis.
Neurology. 1986;36:719–721. [PubMed] [Google Scholar]92. Carmona MA, Jaume Anselmi F, Ramírez Rivera J. Cerebral thrombosis and vasculitis: an uncommon complication of ulcerative colitis. Bol Asoc Med P R. 2000;92:9–11. [PubMed] [Google Scholar]93. Pandian JD, Henderson RD, O’Sullivan JD, Rajah T. Cerebral vasculitis in ulcerative colitis. Arch Neurol. 2006;63:780. [PubMed] [Google Scholar]94. Friol-Vercelletto M, Mussini JM, Bricout JH, Magne C. [Ulcero-hemorrhagic rectocolitis. Possible manifestation, angiitis of the central nervous system] Presse Med. 1984;13:1218. [PubMed] [Google Scholar]95. Druschky A, Heckmann JG, Druschky K, Huk WJ, Erbguth F, Neundörfer B. Severe neurological complications of ulcerative colitis. J Clin Neurosci. 2002;9:84–86. [PubMed] [Google Scholar]96. Masaki T, Muto T, Shinozaki M, Kuroda T. Unusual cerebral complication associated with ulcerative colitis. J Gastroenterol. 1997;32:251–254. [PubMed] [Google Scholar]97. Schluter A, Krasnianski M, Krivokuca M, Spielmann RP, Neudecker S, Hirsch W.
Magnetic resonance angiography in a patient with Crohn’s disease associated cerebral vasculitis. Clin Neurol Neurosurg. 2004;106:110–113. [PubMed] [Google Scholar]99. Holzer K, Esposito L, Stimmer H, Hemmer B, Poppert H. Cerebral vasculitis mimicking migraine with aura in a patient with Crohn’s disease. Acta Neurol Belg. 2009;109:44–48. [PubMed] [Google Scholar]100. Viget N, Vernier-Massouille G, Salmon-Ceron D, Yazdanpanah Y, Colombel JF. Opportunistic infections in patients with inflammatory bowel disease: prevention and diagnosis. Gut. 2008;57:549–558. [PubMed] [Google Scholar]101. Ma C, Walters B, Fedorak RN. Varicella zoster meningitis complicating combined anti-tumor necrosis factor and corticosteroid therapy in Crohn’s disease. World J Gastroenterol. 2013;19:3347–3351. [PMC free article] [PubMed] [Google Scholar]102. Majumder S, Kumar A. Meningococcal meningoencephalitis after certolizumab pegol treatment in a patient with Crohn’s disease. J Crohns Colitis. 2013;7:e19. [PubMed] [Google Scholar]
Brain Lesions: Overview and More
A brain lesion is a type of abnormality in the brain, usually caused by a disease or injury. There are several kinds of brain lesions, and they can be distinguished by their symptoms, physical examination findings, and diagnostic tests.
If you have neurological symptoms, it is important that your doctors determine whether you have a brain lesion, how many you have, and the type because the treatment and prognosis of each type of brain lesion differs.
Rafe Swan / Getty Images
Brain Lesion Symptoms
The effects of brain lesions correspond to the area of the brain where they are located. Usually, the region of the brain where a lesion is located will not function as it normally would.
Symptoms can be gradual or sudden and may be intermittent or constant. Each type of lesion has an emblematic pattern when it comes to the timing of symptoms.
Common symptoms of brain lesions may include a combination of general and focal symptoms.
General Symptoms
General symptoms include:
- Head pain
- Fatigue
- Dizziness
- Behavioral changes
- Cognitive impairment
Focal Neurological Deficits
Focal neurological deficits include:
- Weakness of one side of the face, or the arm and/or leg on one side of the body
- Diminished sensation and/or paresthesias (unusual sensations) on one side of the face, or the arm and/or leg on one side of the body
- Vision changes
- Impaired balance
- Neck stiffness
- Ear pressure
- Seizures
A large brain lesion may cause head pain along with substantial focal neurological deficits (such as weakness of the face, arm, and leg on one side). Multiple lesions tend to cause behavioral changes, cognitive changes, fatigue, and/or dizziness along with one or more focal neurological deficits.
Causes and Types
The different types of brain lesions are each associated with certain causes and risk factors.
Stroke
An interruption in blood flow within the brain can cause a stroke, which is a small or large area of brain damage. Risk factors include heart disease, high blood pressure, uncontrolled diabetes, and high cholesterol. This lesion usually consists of a central area of ischemia (death of cells due to deficient blood supply).
Right after a stroke occurs, the lesion may be surrounded by swelling and inflammation, which subside within a few weeks. The ischemic part of the lesion remains, with permanent damage to the affected area of the brain.
Demyelination
Some conditions, such as multiple sclerosis (MS), are caused by temporary or lasting demyelination—loss of the protective myelin coating that surrounds nerves in the brain. This causes one or more demyelinating brain lesions. The condition is usually idiopathic (without a known cause).
A more serious condition, progressive multifocal leukoencephalopathy (PML), is considered a demyelinating inflammatory response to a virus that is usually harmless. PML generally affects people who are severely immunocompromised, and it has a high mortality rate.
Infection
Bacterial, fungal, viral, or parasitic brain infections can cause one or more areas of damage and inflammation. Many types of brain infections can resolve with appropriate treatment.
Inflammation
Inflammatory lesions in the brain can develop due to conditions like lupus, sarcoidosis, therapeutic brain radiation, and more.
Cancer
Brain tumors and metastatic tumors from elsewhere in the body can cause one or more lesions throughout the brain. The cause of brain tumors is usually unknown. Tumors that metastasize and cause lesions in the brain may have known risk factors. For example, smoking increases the risk of lung cancer, which can spread to the brain.
Vascular Malformations
Vascular malformations such as brain aneurysms and arteriovenous malformations (AVMs) are usually idiopathic. They can be congenital (present from birth) or develop later in life.
These lesions can cause focal neurological deficits when they are small, but they may cause rapid bleeding and severe swelling in the brain if the malformed blood vessels bleed—and could lead to death.
Contusion
Head trauma can lead to a bruise in the brain, which may cause a combination of generalized and focal effects. Contusions in the brain usually partially or fully heal over time. However, repeated blows to the head with multiple lesions can cause chronic traumatic encephalopathy (CTE), with persistent changes.
Hemorrhage
Bleeding in the brain can cause a hemorrhagic lesion. These lesions are more life-threatening than non-hemorrhagic lesions. A number of problems can lead to hemorrhagic lesions, including a bleeding vascular malformation, hemorrhagic conversion of an ischemic stroke, brain tumors that bleed, and head trauma.
Atrophy and Ventricular Dilatation
Sometimes the brain can shrink in size due to damage or dying of the brain cells. This is usually a result of dementia or extensive strokes. This can lead to enlarged spaces within the skull, often noted as atrophic lesions.
Congenital Malformation
Developmental compromise during fetal development can lead to lesions in which areas of the brain are shaped differently than they would be in a healthy brain. Sometimes these malformations lead to impaired physical and cognitive deficits and seizures.
Congenital malformation of the brain can result from genetics or issues such as toxins or insufficient oxygen supply during fetal growth.
Diagnosis
The diagnosis of brain lesions is based on the history and pattern of symptoms, family history, physical examination, neurological examination, and brain imaging.
There are several types of diagnostic brain studies and brain imaging studies, and some can detect certain brain lesions, while others are better at detecting other brain lesions.
For example:
- Brain computerized tomography (CT) is traditionally considered a good test for detecting hemorrhage.
- Brain magnetic resonance imaging (MRI) is considered a good test for detecting demyelination.
- Vascular malformations are often well visualized with diagnostic studies that examine the blood vessels, such as magnetic resonance angiography (MRA).
- Contrast dye helps to define certain lesions, such as tumors and infections.
- Electroencephalogram (EEG) is often used to detect seizure activity caused by brain lesions, some of which cannot be seen on brain imaging studies.
Some lesions, such as demyelinating lesions in MS, can come and go on brain imaging, despite persistent symptoms and physical examination findings. But the condition must be controlled even when lesions are not visibly present. Lesions due to trauma may cause symptoms and physical examination changes with minimal imaging changes.
Brain aneurysms and AVMs might not be visible except with vascular studies focused on the specific area of the lesion. Your doctor would order your imaging study based on the findings of your physical examination—which can consist of very subtle changes in the context of vascular malformations.
Treatment
Brain lesion treatment depends on the cause. Some lesions, such as infections and cancer, can be treated with medication with the goal of a complete cure. Vascular malformations may need to be surgically treated to prevent a rupture. Other lesions, such as demyelination and chronic inflammation, are managed and controlled with medication, but they are not typically curable.
And lesions that cause permanent damage, such as brain contusions, infarct, and hemorrhage, are not curable—but the effects should be managed with rehabilitation. Various types of therapy—physical therapy, speech therapy, cognitive therapy, and more—can help in the recovery and maximizing abilities.
A Word From Verywell
Lesions in the brain usually cause symptoms and can cause lasting damage unless they are treated. Brain lesions can occur for a variety of reasons, and once you know which type of brain lesion you have, you will know what to expect for the long term and the short term.
Modeling the Impact of Lesions in the Human Brain
Abstract
Lesions of anatomical brain networks result in functional disturbances of brain
systems and behavior which depend sensitively, often unpredictably, on the
lesion site. The availability of whole-brain maps of structural connections
within the human cerebrum and our increased understanding of the physiology and
large-scale dynamics of cortical networks allow us to investigate the functional
consequences of focal brain lesions in a computational model. We simulate the
dynamic effects of lesions placed in different regions of the cerebral cortex by
recording changes in the pattern of endogenous
(“resting-state”) neural activity. We find that lesions
produce specific patterns of altered functional connectivity among distant
regions of cortex, often affecting both cortical hemispheres. The magnitude of
these dynamic effects depends on the lesion location and is partly predicted by
structural network properties of the lesion site. In the model, lesions along
the cortical midline and in the vicinity of the temporo-parietal junction result
in large and widely distributed changes in functional connectivity, while
lesions of primary sensory or motor regions remain more localized. The model
suggests that dynamic lesion effects can be predicted on the basis of specific
network measures of structural brain networks and that these effects may be
related to known behavioral and cognitive consequences of brain lesions.
Author Summary
Every year, millions of people suffer the consequences of brain damage, as a
result of stroke, traumatic brain injury, cancer or degenerative brain disease.
The cognitive and behavioral symptoms of focal lesions of the brain are highly
variable and in many cases depend on the location of the lesion site. Can we
predict the functional impact of such lesions on the basis of a computational
model of the brain’s structure and dynamics? Numerous other systems
that form complex networks have been analyzed for their vulnerability to
structural damage. In many cases, the degree to which such systems are perturbed
depends on network attributes of the deleted nodes and connections. We apply
this network approach to investigate the structural and functional impact of
localized lesions of a model of the cerebral cortex. When we delete nodes that
occupy, in the intact brain, a highly central position, we find that the dynamic
interactions between nodes in the remaining brain are greatly disturbed. In
contrast, deletion of less central nodes has relatively little effect. In the
model, some of the most disruptive lesion sites correspond to locations in the
brain where lesions produce complex cognitive disturbances. Our modeling
approach aims towards linking disturbances of structural brain networks to
specific clinical outcomes.
Citation: Alstott J, Breakspear M, Hagmann P, Cammoun L, Sporns O (2009) Modeling the Impact of Lesions in the Human Brain. PLoS Comput Biol 5(6):
e1000408.
https://doi.org/10.1371/journal.pcbi.1000408
Editor: Karl J. Friston, University College London, United Kingdom
Received: March 16, 2009; Accepted: May 6, 2009; Published: June 12, 2009
Copyright: © 2009 Alstott et al. This is an open-access article distributed under the
terms of the Creative Commons Attribution License, which permits unrestricted use,
distribution, and reproduction in any medium, provided the original author and
source are credited.
Funding: OS, MB and JA were supported by the JS McDonnell Foundation. LC was supported by
a grant for interdisciplinary biomedical research by the University of Lausanne.
PH was supported by the Swiss National Science Foundation and the Department of
Radiology of University Hospital Center in Lausanne. The funders had no role in
study design, data collection and analysis, decision to publish, or preparation
of the manuscript.
Competing interests: The authors have declared that no competing interests exist.
Introduction
Recent advances in noninvasive imaging technology have allowed the creation of
comprehensive whole-brain maps of the structural connections of the human cerebrum
[1]–[7]. These maps have led to
the quantitative characterization of various aspects of the network architecture of
the brain, including degree distributions, small-world attributes, centrality and
modularity. Comparative studies of structural and functional connectivity indicate
that the presence of structural links between pairs of cortical regions is
predictive of the occurrence of endogenously driven (resting-state) functional
connectivity [4],[8],[9]. The mapping of
structural connectivity has also enabled the construction of computational models of
resting state activity [10],[11]. The direct comparison of empirically observed
and computationally modeled resting state functional connectivity revealed a high
degree of overlap, supporting the idea that large-scale structural brain networks do
indeed shape and constrain endogenous patterns of functional connectivity [8].
The structural or functional robustness of networks has been investigated in a number
of complex systems [12],[13], including biological networks [14]–[16] In the case of the
brain, acute injuries from trauma, tumor, or stroke, as well as chronic or
degenerative disturbances due to disease, correspond to node and edge deletions in
the structural brain network. Many of the cognitive and behavioral effects of brain
lesions are highly variable and their mechanistic origins remain difficult to
discern. Nevertheless, lesions of specific brain regions are often associated with
specific cognitive and behavioral disturbances, and lesions of some areas tend to
have more severe effects than others [17]–[19].
Vulnerability analyses [20]–[24] of several non-human
primate cortical networks suggest that lesion effects show regional specificity as
well as non-local and distributed effects.
We describe a model of lesion effects in the human brain, based on a previously
published map of structural connections [4] and a biophysical model
of endogenous neural dynamics [8]. We investigate the effects of focal lesions
(removing a spatially localized set of nodes and connections) on the endogenous
dynamics of the remaining brain. We identify structural measures of brain
connectivity that are predictive of the magnitude of the perturbations in the
endogenous neural dynamics. We discuss our results in light of known behavioral and
cognitive lesion effects. The computational and complex network approach taken in
this paper provides a new link between localized structural damage of brain networks
and global disruptions of dynamic interactions.
Methods
Connectivity Data Set
The structural connectivity (SC) data set used in the present paper is identical
to the one described and displayed in ref [8], based on diffusion
MRI data first described in ref [4]. Briefly,
structural connections were derived from diffusion spectrum imaging (DSI) of
five healthy right handed male participants. The segmented cortical gray matter
was partitioned into 66 anatomical regions according to anatomical landmarks
using Freesurfer (surfer.nmr.mgh.harvard.edu) and 998 regions of interest
(ROIs). The 998 ROIs were chosen to provide a roughly uniform tiling of the
cerebral cortex (each ROI∼1.5 cm2) so that their borders
aligned with those of the 66 anatomical regions. White matter tractography was
performed with a custom streamline algorithm and fiber connectivity was
aggregated across all voxels within each of the 998 predefined ROIs. The fiber
strengths produced by the streamline tractography algorithm were exponentially
distributed and spanned several orders of magnitude. Since connection weights in
our model are meant to express physiological efficacy rather than fiber counts
or the thickness of fiber tracts, we resampled the raw fiber strengths into a
Gaussian distribution with a mean of 0.5 and a standard deviation of 0.1
dimensionless units. This transformation does not alter the rank-ordering of
strong to weak pathways, but it compresses the scale of physiological efficacies
(connection strengths). We created an “average SC matrix”
from the resampled connection maps of individual participants. In this average
SC map, structural connections were deemed absent overall, i.e. set to zero, if
they were absent in more than 3 participants.
Modeled Neural Dynamics
Neuronal dynamics were simulated using a system of neural masses coupled to one
another with strengths linearly proportional to the resampled fiber strengths at
each edge. Each neural mass represents a population of densely interconnected
excitatory and inhibitory neurons, in which the effects of both ligand- and
voltage-gated membrane channels are accounted for. This model was first
developed in [25] and has previously been employed in an
anatomically-informed model of large-scale functional connectivity in the
macaque monkey [10] as well as for modeling human resting-state
functional connectivity [8]. The model was simulated in Matlab R2007a
(Mathworks, Natick, MA) at a time resolution of 0.2 msec. Before data analysis,
resulting data sets are downsampled to a time resolution of 1 millisecond. After
an initial transient of 2 minutes which was discarded, runs proceeded for a
total of 8 minutes. Simulated BOLD signals were computed by using a nonlinear
hemodynamic model as previously described [8],[10],[26].
While all simulations were carried out with the same set of haemodynamic
parameters, future studies may incorporate individual variations, e.g. to take
into account effects of disease state on blood vessel compliance, or regional
variations of the haemodynamic response across different brain regions.
Cross-correlation matrices of BOLD time-series (functional connectivity, FC)
were derived without regressing out the global signal average, as this procedure
may affect correlation pattern and magnitude. For each lesion, as well as for
unlesioned controls, we conducted five simulation runs starting from random
initial conditions. Data analyses were carried out on correlation matrices
averaged over these five runs. For more details see refs. [8],[10],[25].
Lesions
The structural connectivity matrix was lesioned in two ways: sequential single
node deletions and localized area removal. The first method was aiming at a
structural failure analysis, and included both “random” and
“targeted” node deletions, involving the sequential removal
of nodes (ROIs), one by one, until the network had shrunk to a single remaining
node. For random node removal, we removed a single randomly chosen node at each
step. This process was repeated 25 times. For targeted node removal, we first
computed the node degree (defined as the number of connections at each node),
node strength (defined as the sum of all the weights of the connections at each
node) or the node betweenness centrality [27] for all nodes in
the network. Then we removed the single node with the highest degree, strength
or centrality. Degree, strength and centrality were then re-computed and the
next node was selected for removal, until one last node remained. At each step
during random and targeted node removal we calculated several structural network
measures, including the size of the largest connected component of the remaining
network and the global efficiency. Global efficiency is computed as the average
of the inverse distance between all nodes and captures the network’s
capacity for communication along short paths [28].
The second lesion type, localized lesions, was aiming at dynamic and functional
failure analysis. These lesions were carried out by removing all nodes and their
connections within a spatially defined region around a central location. The
central location was defined by a standard x,y,z Talairach coordinate and a
fixed number of ROIs closest to this central location were deleted. Closeness
was determined by the Euclidean distance. Lesions involved the deletion of nodes
(“gray matter”) and their afferent/efferent connections only
– we did not attempt to model “white matter”
volume, for example by including lesions of “fibers of
passage”. Computational considerations prevented us from simulating
lesions centered on all 998 ROIs, and from varying the lesion extent. We
selected a lesion size of 50 ROIs, corresponding to about 5% of the
cortical surface, which was large enough to have significant effects on neural
dynamics, and small enough to preserve the regional specificity of the lesions.
A complete list of all lesions, their central locations, spatial coordinates,
and affected anatomical subregions are provided in Table 1. The spatial location and extent of
all lesions is depicted in Figure
1. Jointly, all lesions described in this paper cover about 80 percent of
the cortical surface. Figure
1 also illustrates the relation of all lesions to the default mode
network (DMN). The DMN was comprised of 200 ROIs which had earlier been
determined from empirical fMRI studies [8], and contained
portions of the precuneus/posterior cingulate cortex, medial and superior
frontal cortex, and lateral parietal cortex.
Figure 1. Lesion locations.
Diagrams show a rendering of a standard cortical surface, with ROIs that
form part of the DMN indicated in light red. Outlines indicate
approximate lesion locations. All lesions are comprised of 50 ROIs.
Lesion labels correspond to lesion names in Table 1 and 2.
https://doi.org/10.1371/journal.pcbi.1000408.g001
All graph-theoretical measures (path length, centrality, efficiency) reported in
this study were computed from a structural network that preserved edge weights,
as previously described [4].
Measures of Lesion Effects
The nature of the computational model does not allow us to probe directly for
behavioral or cognitive lesion effects. Thus, our measures of lesion effects are
confined to estimates of the lesion’s immediate structural and dynamic
impact. Examples of structural (SC) and BOLD cross-correlation matrices (FC)
before and after a lesion are shown in Figure 2. Lesion effects were quantified in
several ways, all of which produced similar patterns of results (Table 2). The distance
between the unlesioned and lesioned FC matrix was calculated asWhere rij is the functional connectivity measure
(cross-correlation) between nodes i and j. This distance dFC was computed for
both the high-resolution FC matrices (998 ROIs) and for the regionally averaged
FC matrix (66 regions). We computed two distances, one of which included
functional connections of all ROIs (dFC), while the other only measured the
distance between ROI pairs that were not involved in the lesion itself
(dFC′).
Figure 2. Structural connectivity, functional connectivity, and measurement of
lesion effects.
(A) Top: Intact “unlesioned” structural connectivity
(SC). Bottom: lesioned SC. The lesion shown here is L194 and the
lesioned portion of the matrix is indicated in light yellow. (B) Top:
Unlesioned functional connectivity (FC) matrix, obtained after averaging
BOLD cross-correlations from 5 simulation runs. Bottom: lesioned FC
matrix (L194), averaged over 5 runs. (C) z-score matrix after
subtraction of normalized cross-correlations. (D) Cumulative
distribution of z-scores of functional connections after subtraction of
lesioned (L194) from unlesioned FC (blue dots) and after subtraction of
two sets of 5 unlesioned runs (black dots). The dashed line marks
z = 3.3, and the number of functional
connections at this threshold was taken as one measure of lesion
impact.
https://doi.org/10.1371/journal.pcbi.1000408.g002
A second way to measure the difference between two correlation matrices was
computed as follows. First, we converted the two correlation matrices (before
and after lesioning) to a normal distribution by using Fisher’s
z-transform. To test the hypothesis that the two sets of correlations were drawn
from different distributions we computed z-scores, according towhere df corresponds to the effective degrees of freedom. The
value for df was estimated following procedures used for analyzing empirically
obtained correlation matrices (e.g. ref [29]). Using a
correction factor for independent frames (estimated according to
Bartlett’s theory [30]) of 3, and computing correlations from 5
independent runs of 8 minutes each, with 30 data samples/minute, we obtained
df = 400. We then counted the number of
functional connections that exceeded a significance threshold of |z|>3.3.
To test the validity of this threshold we compared two correlation matrices
computed from independent sets of 5 unlesioned runs against each other. After
normalization, z-score transformation and thresholding at |z|>3.3, we
detected 91 false positives out of nearly 500,000 comparisons (Figure 2D), indicating that
the error rate is p<0.001. We concluded that for simulations of lesions
the occurrence of a large number of functional connections with |z|>3.3
reflected specific lesion effects with very high probability. Choosing higher
thresholds (e.g. |z|>5) did not affect the main conclusions of the study
(data not shown).
Results
Several previous studies have examined the direct effects of node deletions on
network structure and connectivity. Thus, we first examined the effects of random
and targeted node removal on the structural integrity of the network, measured as
the size of the largest connected component (Figure 3). Random removal of nodes did not affect
network integrity until almost all of the nodes had been deleted. Targeted removal
of nodes on the basis of node degree or node strength disconnected the network only
after approximately three quarters of all nodes had been deleted. In contrast,
targeting nodes on the basis of their centrality resulted in the appearance of
disconnected components after deletion of only 164 nodes. Targeting highly central
nodes also resulted in a rapid decrease in the network’s global efficiency,
while targeted removal of nodes with high degree or high strength resulted in a more
gradual decline in efficiency.. We performed identical analyses on a set of control
networks whose global topology had been randomized while preserving the sequence of
node degrees. These randomized controls were highly resilient to removal of nodes
based on centrality or strength, remaining strongly connected until more than 700
nodes had been deleted (results not shown). These results indicate that the
structural network is relatively insensitive to random node deletion, or to node
deletion targeting nodes according to their degree or strength, while showing much
greater vulnerability to targeted node deletion on the basis of centrality.
Figure 3. Analysis of robustness on the basis of random/targeted node deletions.
The plots show the size of the largest network component (A) and the global
efficiency (B) as a function of the number of deleted nodes. The curve for
random node deletion is an average of 25 different random sequences. The
other three curves represent unique sequences of node deletion determined by
node degree (blue) strength (green) or node centrality (red).
https://doi.org/10.1371/journal.pcbi.1000408.g003
The potential dynamic effects of focal brain lesions on neural activity have remained
relatively unexplored. Here, we compared functional connectivity patterns due to
endogenous neural dynamics before and after a lesion was made. Despite equal lesion
size (50 nodes) dynamic lesion effects exhibited marked differences depending on
lesion location. These differences involved both the magnitude and the spatial
pattern of changed functional connections (Table 2). Posterior and anterior lesions along
the cortical midline, as well as a subset of lesions in frontal, parietal and
temporal cortex, had extensive effects. With few exceptions lesion effects were
stronger in the ipsilateral hemisphere, and mostly involved weakening of functional
coupling. Lesions closer to the midline tended to be more disruptive of
cross-hemispheric coupling than more lateral lesions. A subset of lesions in frontal
cortex and in the anterior cingulate had disproportionately strong effects on
functional connections involving the default mode network.
Figures 4, 5 and 6 show the spatial distribution of functional
connections that exhibited significant differences for a selection of lesion
locations many of which were highly impactful overall, including lesions along the
cortical midline (Figure 4), the
temporo-parietal junction (Figure
5) and the frontal cortex (Figure 6). Other lesions altered functional connectivity less, for
example lesions in primary sensory and motor regions (Figure S1).
Figure 4. Dynamic effects of lesions along the brain’s midline.
(A) L194. (B) L821. In this plot, as well as in Figures 5, 6 and S1, a
dorsal view of the brain (middle panel) and two lateral views of the left
hemisphere (left panels) and the right hemisphere (right panels) are shown.
The middle panel plots all significantly different functional connections,
while the left and right panels only show significantly different functional
connections within the left and right hemispheres, respectively. The 998 ROI
z-score FC matrix was aggregated to 66 subregions, and pathways between
these 66 subregions are plotted if at least 10% of their
constituent connections linking ROI pairs are significantly changed
(|z|>3.3) as a result of the lesion. Pathways are plotted in red or
blue, if their coupling has been weakened or strengthened, respectively. The
approximate lesion center is marked with a green
“+”.
https://doi.org/10.1371/journal.pcbi.1000408.g004
Lesions along the cortical midline were characterized by widespread effects involving
both cerebral hemispheres and all major cortical lobes. L194 (Figure 4A), centered in the right caudal anterior
cingulate cortex resulted in lower functional connectivity between most ipsilateral
subregions of right medial cortex, extending from orbitofrontal cortex to the
cuneus. Some functional connections along the contralateral midline were also
weakened, but to a lesser extent. Interhemispheric functional connections were
profoundly suppressed. Lesions placed in the posterior medial cortex, e.g. L821
(Figure 4B) also affected
functional connectivity in both hemispheres but had less widespread effects.
Contralateral effects consisted of increasing coupling between several regions,
including between superior parietal and anterior cingulate cortex.
Lesions near the temporo-parietal junction were highly disruptive of functional
connectivity within their own cortical hemisphere as well as between hemispheres.
L472 (Figure 5A) was centered in
right superior temporal cortex and resulted in sharply lowered functional
connectivity among all subdivisions of the ipsilateral parietal and posterior
temporal cortex. In addition, coupling between regions in posterior medial cortex
and frontal cortex were decreased in both hemispheres. A lesion in the left inferior
parietal cortex in the vicinity of the left angular gyrus (L810, Figure 5B) significantly increased
functional coupling within the left hemisphere, while suppressing cross-hemispheric
functional connectivity.
Lesions involving parts of frontal cortex resulted in pronounced and widespread loss
of functional coupling within the lesioned hemisphere as well as across hemispheres.
A lesion of right superior frontal cortex (L86, Figure 6A) strongly reduced functional coupling
of many right hemispheric brain regions, including interactions between frontal,
temporal, and parietal cortex, extending over the entire length of the
anterior-posterior axis. Weaker, but significant, suppression of functional
connectivity is also seen in the contralateral hemisphere, including reduced
coupling between the posterior cingulated/precuneus and the superior and middle
frontal cortex. Lesioning left lateral frontal cortex centering on the pars
opercularis (L555, Figure 6B)
reduces functional coupling more locally.
Lesions of primary sensory and motor cortices (Figure S1)
leave the functional connectivity of the remainder of the brain largely unchanged.
Lesions centered in visual cortex (L360) or somatomotor cortex (L162) have little
effect on functional connectivity outside of the immediate vicinity of the lesion
itself.
In addition to node removal, lesions may be modeled as edge deletions, i.e.
disruptions of white matter pathways. One of the most dramatic examples is the
complete transection of the corpus callosum. We performed simulations after deleting
all cross-hemispheric connections and compared the resulting functional connectivity
patterns to those obtained from the intact brain (Figure S2). In
the model, callosal transection resulted in the complete loss of all
inter-hemispheric functional connectivity, as well as a more restricted pattern of
significant changes in intra-hemispheric functional coupling.
Finally, we examined whether the extent of dynamic lesion effects could be predicted
on the basis of the impact of the lesion on structural network measures.
Specifically, we asked if dynamic lesion effects were more pronounced if the lesion
lengthened network paths, removed a larger number of long-range connections, or
removed more highly connected or more highly central nodes. Table 3 and Figure 7 summarize the relationship between these
structural measures and several measures of the dynamic impact of the lesion. The
reported correlations are calculated for a subset of 22 lesion sites covering about
80 percent of the cortical surface, and for a single lesion size (50 nodes). The
extent of dynamic lesion effects was only weakly predicted
(r≈0.4–0.5) by the degree or strength of the nodes within each
lesion. A better predictor was the number of connections between the lesion site and
the rest of the brain (these connections are lost as a result of the lesion), and
how much the lesion increased the path length of the remaining network
(r≈0.45–0.7). Node and edge centrality of the lesioned nodes or
edges predicted functional lesion impact about equally well
(r≈0.45–0.7). The most robust prediction was made by the extent to
which the lesion damaged the default mode network (r≈0.6–0.85).
Figure 7. Summary diagram of relationships between structural lesion measures and
dynamic lesion effects.
Structural lesion measures are the sum of the node strengths of the lesion
(“lesion strength”), the sum of the node centrality of
the lesion (“lesion centrality”) and the extent to which
the lesion included nodes within the DMN. Dynamic lesion effects are the
number of significantly changed functional connections (outside of the
lesioned nodes) and the distance between lesioned and unlesioned FC. Compare
r-values to those in Table
3. * = p<0.05,
** = p<0.01,
*** = p<0.001.
https://doi.org/10.1371/journal.pcbi.1000408.g007
Discussion
The availability of whole-brain structural connectivity data sets [3]–[7], for the first time,
allows for the computational study of the effects of localized structural lesions on
neural dynamics. In this study, lesions are modeled as structural perturbations with
specific dynamic effects. We find that lesions in different regions of the cerebral
cortex have specific effects on the pattern of endogenous functional connectivity of
the remaining brain that differ in both extent and spatial pattern. Generally,
lesions along the cortical midline, the temporo-parietal junction and the frontal
cortex result in the largest and most widespread effects on functional connectivity.
Many lesions affect the functional coupling of brain regions outside of the lesion
itself, including effects in the hemisphere contralateral to the lesion site.
The first part of our study involved random and targeted node deletions and their
impact on the structural integrity of the network (Figure 3). Some of our results expanded upon
observations made by other investigators who examined the vulnerability or
robustness of brain networks [21]–[23]. Our structural network
is relatively resilient against random node removal and against targeting of nodes
on the basis of their high degree or high strength, a finding also reported for
human functional networks [21]. However, the network is much less well protected
against loss of nodes that are highly central, a finding that is consistent with the
overall network architecture which consists of modules linked by hubs [4].
Targeted node removal by centrality may have a physiological basis. There is a
potential link between node centrality and baseline metabolic activity [4] and it
has been suggested that a high rate of metabolism may render neurons vulnerable to
neurodegenerative processes [31],[32]. We hypothesize that at least some forms of
degenerative brain disease may involve the “targeted” removal of
network components.
Confirming earlier results obtained from a much smaller connection matrix of macaque
cortex [24], modeling lesions in the human brain resulted in
non-local dynamic effects. Several empirical studies have demonstrated such
non-local effects, for example changes a distributed pattern of functional
connectivity following in patients with focal brain lesions due to tumor or stroke
[33]–[35]. Early theoretical
accounts had predicted and attempted to explain such nonlocal effects, invoking
concepts such as “diaschisis” [36] or
“disconnection” [37]. The complex network
approach adopted in this paper supports these concepts and provides a new
opportunity to establish links between physical brain damage and functional
disturbances. As suggested by studies of structural network measures [23],[38],
including our own results regarding the effects of targeted node removal (Figure 3), we found that dynamic
lesion effects were particularly large and widespread when lesions included nodes or
edges of high centrality (Figure
7, Table 3). Another
good predictor of functional change was the number of connections between the lesion
site and the rest of the brain that were lost. This result underscores that
“disconnection” may occur not only for areas that are directly
anatomically linked but also account for changes in dynamic coupling among remote
and structurally unconnected areas of cortex. Dynamic lesion effects were especially
pronounced for several highly connected hub nodes within the brain’s
default mode network, for example in medial parietal and cingulate cortex. We
believe that this result applies generally to the type of network and neural
dynamics investigated here, and will hold even as the human connectome [39]
continues to be refined.
The significant computational requirements involved in conducting large-scale
simulations of endogenous brain activity necessitated we limit our analysis to a set
of brain lesions selected for their neurological interest (Figure 1, Table 1). In the model, lesions of regions along
the cortical midline were particularly disruptive. In patients, lesions of posterior
medial cortex (in the vicinity of L323 and L821) are described as rare but resulting
in profound disorders of consciousness [40], while lesions of the
anterior cingulate cortex result in severe disruptions of personality and emotional
processing, apathy and inattention [41]. In the model, lesions centered on the
temporo-parietal junction also resulted in widespread changes in functional
coupling. Empirically, the left angular gyrus (near L810) has been implicated in
dyslexia [42], while lesions centered on the posterior portion of
the right superior temporal cortex (near L472) often result in spatial hemineglect
[43].
In contrast to these large effects of midline and temporo-parietal lesions, modeled
lesions of primary visual and somatomotor cortex had little effect outside of their
respective target regions. In patients, lesions of visual cortex or motor cortex
result in deficits that are severe, but largely limited to loss of function within a
specific modality. While our study does not provide complete coverage of all
possible lesion sizes and locations in cortex we note that the magnitude and
dispersion of the lesion’s dynamic impact is correlated with the clinically
observed severity and range of cognitive deficits.
In the current model we did not attempt to include the effects of lesions of brain
nodes on white matter “fibers of passage”, and neither did we
attempt to systematically explore the functional impact of disruptions of specific
white matter pathways. We provided a single example of fiber damage by modeling the
effects of cutting all inter-hemispheric connections (Figure S2). The
observed pattern matches empirical observations of a striking loss of
inter-hemispheric functional connectivity immediately following callosotomy in a
human patient [29]. Contrasting this observation, residual
functional connectivity between the two cerebral hemispheres observed in a patient
several decades after a complete commissurotomy [44] may be due to
inter-hemispheric coupling via subcortical pathways. An extension of the current
model to include subcortical nodes and connections may provide a structural basis
for the long-term restoration of inter-hemispheric functional connectivity following
callosal transection.
At the present stage, the model cannot be tested for behavioral or cognitive
deficits. While future studies may include a quantitative evaluation of the
structure of pre-/post-lesion effective brain networks resulting from specific
task-related perturbations, here we relied exclusively on the pattern of endogenous
neural dynamics to measure dynamic lesion impact. These endogenous dynamics may be
viewed as a proxy for the cortical “resting state” in the human
brain [45],[46], which has been shown
to be disrupted or altered in the course of disease states [31]. For example, changes
in resting-state activation and functional connectivity may serve as diagnostic
markers for the onset, progression or severity of Alzheimer’s disease [32] and
schizophrenia [47],[48]. Both conditions are known to be associated with
disturbances of structural brain connectivity, including portions of the default
mode network. Here, we observed that lesions that included portions of the default
mode network had particularly large and widespread effects on functional
connectivity throughout the brain. This is consistent with previously observed
strong structural and functional coupling among ROIs in the DMN [8] and its
association with major hubs in the cortex [4],[32]. Our model suggests
that the pattern of endogenous neural activity, in particular within the default
mode network, may serve as a marker of the degree of functional disturbance. A
further implication is that the restoration of the topology of resting-state
functional connectivity may aid in cognitive repair and recovery [49].
The structural connectivity pattern used in the present model was obtained by
noninvasive diffusion imaging [4]. Future mapping studies of the human connectome
will likely provide improved imaging and reconstruction of crossing, highly curved,
or long-distance fiber pathways, thus providing a more accurate structural model. In
addition, several current limitations of the model should be addressed: a) The model
contains only cerebral cortical regions and pathways, and does not account for
axonal conduction delays; b) The model does not take into account white matter
damage of “fibers of passage” in addition to node deletion; c)
The model captures only immediate lesion effects without including mechanisms of
neural plasticity which may support reorganization and functional recovery. We
believe these limitations can be overcome as available data sets and computational
modeling tools improve. A particularly fruitful avenue for future work is the
incorporation of longitudinal data on structural and functional processes following
acute brain injury. The further development of noninvasive imaging technology in
combination with sophisticated computational modeling may eventually allow the
design of individualized treatment and recovery protocols that help improve
behavioral outcomes following acute cortical lesions.
Supporting Information
Figure S2.
Dynamic effects of the complete transection of all interhemispheric
connections (corpus callosum). The panel on the left shows the intact
pattern of functional connectivity, estimated from a seed region located
near the right hemispheric frontal eye fields at [28, -7,
54], matching the seed location in Figure 2 of ref. [29]. The intact
pattern shows positive coupling between frontal and parietal cortex, as well
as between homologous structures in the two hemispheres. The panel on the
right shows the pattern of functional connectivity, again seeded at
[28, -7, 54], after complete transection of all callosal
connections. Interhemispheric functional connections are abolished, while
intrahemipsperic functional connections are largely preserved.
https://doi.org/10.1371/journal.pcbi.1000408.s002
(5.05 MB TIF)
Author Contributions
Conceived and designed the experiments: JA MB PH OS. Performed the
experiments: JA OS. Analyzed the data: JA OS. Contributed
reagents/materials/analysis tools: JA MB PH LC OS. Wrote the paper: JA
OS.
References
- 1.
Johansen-Berg H, Behrens TEJ (2009) Diffusion MRI: From Quantitative Measurement to in vivo Neuroanatomy. London: Academic Press. - 2.
Bullmore ET, Sporns O (2009) Complex brain networks: graph-theoretical analysis of structural
and functional systems. Nature Rev Neurosci 10: 186–198. - 3.
Hagmann P, Kurant M, Gigandet X, Thiran P, Wedeen VJ, et al. (2007) Mapping human whole-brain structural networks with diffusion MRI. PLoS ONE 2: e597. - 4.
Hagmann P, Cammoun L, Gigandet X, Meuli R, Honey CJ, Wedeen VJ, Sporns O (2008) Mapping the structural core of human cerebral cortex. PLoS Biol 6: e159. - 5.
Iturria-Medina Y, Sotero RC, Canales-Rodriguez EJ, Aleman-Gomez Y, Melie-Garcia L (2008) Studying the human brain anatomical network via
diffusion-weighted MRI and graph theory. NeuroImage 40: 1064–1076. - 6.
Gong G, He Y, Concha L, Lebel C, Gross DW, Evans AC, Beaulieu C (2009) Mapping anatomical connectivity patterns of human cerebral cortex
using in vivo diffusion tensor imaging tractography. Cereb Cortex 19: 524–536. - 7.
Perrin M, Cointepas Y, Cachia A, Poupon C, Thirion B, et al. (2008) Connectivity-based parcellation of the cortical mantle using
q-ball diffusion imaging. Int J Biomed Imaging 368406. - 8.
Honey CJ, Sporns O, Cammoun L, Gigandet X, Thiran JP, Meuli R, Hagmann P (2009) Predicting human resting-state functional connectivity from
structural connectivity. Proc Natl Acad Sci U S A 106: 2035–2040. - 9.
Skudlarski P, Jagannathan K, Calhoun VD, Hampson M, Skudlarska BA, Pearlson G (2008) Measuring brain connectivity: Diffusion tensor imaging validates
resting state temporal correlations. NeuroImage 43: 554–561. - 10.
Honey CJ, Kötter R, Breakspear M, Sporns O (2007) Network structure of cerebral cortex shapes functional
connectivity on multiple time scales. Proc Natl Acad Sci U S A 104: 10240–10245. - 11.
Ghosh A, Rho Y, McIntosh AR, Kotter R, Jirsa VK (2008) Noise during rest enables the exploration of the brain’s
dynamic repertoire. PLoS Comput Biol 4: e1000196. - 12.
Albert R, Jeong H, Barabási AL (2000) Error and attack tolerance of complex networks. Nature 406: 378–382. - 13.
Doyle JC, Alderson DL, Li L, Low S, Roughan M, et al. (2005) The “robust yet fragile” nature of the
internet. Proc Natl Acad Sci U S A 102: 14497–14502. - 14.
Jeong H, Mason SP, Barabási AL, Oltvai ZN (2001) Lethality and centrality in protein networks. Nature 411: 41–42. - 15.
Barabási AL, Oltvai ZN (2004) Network biology: understanding the cell’s functional
organization. Nat Rev Genet 5: 101–113. - 16.
Proulx S, Promislow DEL, Phillips PC (2005) Network thinking in ecology and evolution. Trends Ecol Evol 20: 345–353. - 17.
Damasio H, Damasio AR (1989) Lesion Analysis in Neuropsychology. New York: Oxford University Press. - 18.
Mesulam MM (2000) Principles of Behavioral and Cognitive Neurology. Oxford: Oxford University Press. - 19.
Bogousslavsky J, Caplan L (2001) Stroke Syndromes. Berlin: Springer. - 20.
Young MP, Hilgetag CC, Scannell JW (2000) On imputing function to structure from the behavioural effects of
brain lesions. Phil Trans R Soc Lond B Biol Sci 355: 147–161. - 21.
Achard S, Salvador R, Whitcher B, Suckling J, Bullmore ET (2006) A resilient, low-frequency, small-world human brain functional
network with highly connected association cortical hubs. J Neurosci 26: 63–72. - 22.
Kaiser M, Robert M, Andras P, Young MP (2007) Simulation of robustness against lesions of cortical networks. Eur J Neurosci 25: 3185–3192. - 23.
Kaiser M, Hilgetag CC (2004) Edge vulnerability in neural and metabolic networks. Biol Cybern 90: 311–317. - 24.
Honey CJ, Sporns O (2008) Dynamical consequences of lesions in cortical networks. Hum Brain Mapp 29: 802–809. - 25.
Breakspear M, Terry J, Friston K (2003) Modulation of excitatory synaptic coupling facilitates
synchronization and complex dynamics in a biophysical model of neuronal
dynamics. Network: Computation in Neural Systems 14: 703–732. - 26.
Friston K, Mechelli A, Turner R, Price C (2000) Nonlinear responses in fMRI: The balloon model, Volterra kernels,
and other hemodynamics. NeuroImage 12: 466–477. - 27.
Freeman LC (1977) A set of measures of centrality based on betweenness. Sociometry 40: 35–41. - 28.
Latora V, Marchiori M (2001) Efficient behavior of small-world networks. Phys Rev Lett 87: p198701. - 29.
Johnston JM, Vaishnavi SN, Smyth MD, Zhang D, He BJ, et al. (2008) Loss of resting interhemispheric functional connectivity after
complete section of the corpus callosum. J Neurosci 28: 6453–6458. - 30.
Jenkins GM, Watts DG (1968) Spectral Analysis and its Applications. San Francisco: Holden-Day. - 31.
Buckner RL, Andrews-Hanna JR, Schacter DL (2008) The brain’s default network: anatomy, function, and
relevance to disease. Ann N Y Acad Sci 1124: 1–38. - 32.
Buckner RL, Sepulchre J, Talukdar T, Krienen FM, Liu H, et al. (2009) Cortical hubs revealed by intrinsic functional connectivity:
Mapping, assessment of stability, and relation to Alzheimer’s
disease. J Neurosci 29: 1860–1873. - 33.
Corbetta M, Kincade MJ, Lewis C, Snyder AZ, Sapir A (2005) Neural basis and recovery of spatial attention deficits in
spatial neglect. Nature Neurosci 8: 1603–1610. - 34.
He BJ, Snyder AZ, Vincent JL, Epstein A, Shulman GL, et al. (2007) Breakdown of functional connectivity in frontoparietal networks
underlies behavioral deficits in spatial neglect. Neuron 53: 905–918. - 35.
Bartolomei F, Bosma I, Klein M, Baayen JC, Reijneveld JC, et al. (2006) Disturbed functional connectivity in brain tumour patients:
Evaluation by graph analysis of synchronization matrices. Clin Neurophysiol 117: 2039–2049. - 36.
von Monakow C (1914) Die Lokalisation im Grosshirn und der Abbau der Funktion durch kortikale
Herde. Wiesbaden: JF Bergmann. - 37.
Geschwind N (1965) Disconnexion syndromes in animals and man. Brain 88: 237–94. - 38.
Sporns O, Honey CJ, Kötter R (2007) Identification and classification of hubs in brain networks. PLoS ONE 2: e1049. - 39.
Sporns O, Tononi G, Kötter R (2005) The human connectome: A structural description of the human
brain. PLoS Comp Biol 1: e42. - 40.
Damasio A (1999) The Feeling of What Happens. New York: Harcourt Brace. - 41.
Bush G, Luu P, Posner MI (2000) Cognitive and emotional influences in anterior cingulate cortex. Trends Cogn Sci 4: 215–222. - 42.
Horwitz B, Rumsey JM, Donohue BC (1998) Functional connectivity of the angular gyrus in normal reading
and dyslexia. Proc Natl Acad Sci U S A 95: 8939–8944. - 43.
Karnath HO, Ferber S, Himmelbach M (2001) Spatial awareness is a function of the temporal not the posterior
parietal lobe. Nature 411: 950–953. - 44.
Uddin LQ, Mooshagian E, Zaidel E, Scheres A, Margulies DS, et al. (2008) Residual functional connectivity in the split-brain revealed with
resting-state functional MRI. NeuroReport 19: 703–709. - 45.
Raichle ME, MacLeod AM, Snyder AZ, Powers WJ, Gusnard DA, Shulman GL (2001) A default mode of brain function. Proc Natl Acad Sci U S A 98: 676–682. - 46.
Greicius MD, Krasnow B, Reiss AL, Menon V (2003) Functional connectivity in the resting brain: a network analysis
of the default mode hypothesis. Proc Natl Acad Sci U S A 100: 253–258. - 47.
Whitfield-Gabrieli S, Thermenos HW, Milanovic S, Tsuang MT, Faraone SV, et al. (2009) Hyperactivity and hyperconnectivity of the default network in
schizophrenia and in first-degree relatives of persons with schizophrenia. Proc Natl Acad Sci U S A 106: 1279–1284. - 48.
Rubinov M, Knock SA, Stam CJ, Micheloyannis S, Harris AWF, Williams LM, Breakspear M (2009) Small world properties of nonlinear brain activity in
schizophrenia. Human Brain Mapping 30: 403–416. - 49.
Rubinov M, McIntosh AR, Valenzuela MJ, Breakspear M (2008) Simulation of neuronal death and network recovery in a
computational model of distributed cortical activity. Am J Geriatr Psychiatry PMID 19001355.
Brain lesion locations associated with secondary seizure generalization
Abstract
Background Structural brain lesions are the most common cause of adult-onset epilepsy. The lesion location may contribute to the risk for epileptogenesis, but whether specific lesion locations are associated with a risk for secondary seizure generalization from focal to bilateral tonic-clonic seizures, is unknown.
Methods We identified patients with a diagnosis of adult-onset epilepsy caused by an ischemic stroke or a tumor diagnosed at the Turku University Hospital in 2004-2017. Lesion locations were segmented on patient-specific MR imaging and transformed to a common brain atlas (MNI space). Both region-of-interest analyses (intersection with the cortex, hemisphere, and lobes) and voxel-wise analyses were conducted to identify the lesion locations associated with focal to bilateral tonic-clonic compared to focal seizures.
Results We included 170 patients with lesion-induced epilepsy (94 tumors, 76 strokes). Lesions predominantly localized in the cerebral cortex (OR 2.50, 95% C.I. 1.21-5.15, p = 0.01) and right hemisphere (OR 2.22, 95% C.I. 1.17-4.20, p = 0.01) were independently associated with focal to bilateral tonic-clonic seizures. At the lobar-level, focal to bilateral tonic-clonic seizures were associated with lesions in the right frontal cortex (OR 4.41, 95% C.I. 1.44-13.5, p = 0.009). No single voxels were significantly associated with seizure type. These effects were independent of lesion etiology.
Conclusions Our results demonstrate that lesion location is associated with the risk for secondary generalization of epileptic seizures independent of lesion etiology. These findings may contribute to identifying patients at risk for focal to bilateral tonic-clonic seizures.
Introduction
Epilepsy is the fourth most common neurological disease, affecting more than 20 million people of all ages worldwide.1,2 Brain lesions are the most common cause of adult-onset epilepsy.3 Although almost all brain lesions can cause epilepsy, some types of lesions, such as infiltrating tumors, are more prone to cause epilepsy than other types, such as stroke.3,4 Clinical risk factors for lesion-induced epilepsy vary depending on the lesion etiology but on a patient level it’s challenging to predict which lesions are likely to cause epilepsy and which are not.5,6
Lesion location has been suggested to play a role in the development of lesional epilepsy.4-6 In general, lesions in the cerebral cortex are associated with higher risk for epilepsy than lesions in other parts of the brain.4,5,7,8 In stroke, lesions in the anterior circulation area are associated with increased risk for late seizures and epilepsy compared to strokes in the posterior circulation.5,7 In gliomas, tumor locations in the temporal and frontal lobe have been linked to an increased risk of seizures. However, there is no clear consensus between studies.4,8-10 Given that focal seizures can theoretically arise from any brain region, the lack of unifying brain region associated with epilepsy across lesion etiologies is not surprising.
Lesion-induced epilepsy is considered to cause focal epileptic activity, which in some patients spreads to other brain regions outside the focus and may eventually lead to bilateral tonic-clonic seizures (focal to bilateral tonic-clonic, FTBTC).11 Secondary seizure generalization in FTBTC seizures involves cortical spreading of epileptic activity to both hemispheres and is hypothesized to include spread to subcortical brain regions including the thalamus, basal ganglia and cerebellum.12 However, the underlying mechanisms and pathways leading to FTBTC seizures are still unclear and it is not known if the anatomical lesion location plays a role in the generalization of seizures.13 FTBTC seizures are associated with a loss in quality of life2, a high risk of injury14, and an increased risk for sudden unexpected death in epilepsy (SUDEP).15 Identifying lesion locations associated with increased risk for FTBTC seizures may help to identify these patients at an early stage in treatment and benefit patient-specific epilepsy management. Moreover, characterizing lesion locations associated with FTBTC seizures may be valuable for understanding the mechanisms of secondary seizure generalization.11,16,17
The aim of this study was to investigate if lesion locations are associated with FTBTC seizures across stroke and astrocytoma, two common lesion etiologies associated with epilepsy. Using both a-priori-region of interest analyses and data-driven voxel-wise analyses, we compared lesion locations of patients FTBTC seizures to patients with focal seizures only.
Materials and methods
Patient selection
Patients with lesion-induced epilepsy were identified from clinical records between 2004 and 2017 at Turku University Hospital, Turku, Finland. A systematic database search for patients with age >/=18 years with diagnosis of symptomatic epilepsy (International Classification of Diseases 10, ICD-10 codes: G40.00, G40.01, G40.09, G40.10, G40.11, G40.12, G40.19, G40.20, G40.21, G40.22 and G40.29) and brain MRI obtained up to +/-3 months from the diagnosis of epilepsy was conducted. A relatively short interval between the epilepsy diagnosis and brain MRI was chosen to minimize the possibility of additional brain lesions or other confounding factors after the occurrence of the lesion. This search identified 1887 patients who were selected for a detailed clinical record review.
The case selection is illustrated in Figure 1. The clinical diagnoses and seizure types were retrieved from the hospital records. All epilepsy diagnoses were established at the department of neurology at the Turku University Hospital. All relevant clinical records including neuroimaging and clinical neurophysiology examination results and clinical follow up were carefully evaluated by the investigators. Out of 1887 cases evaluated, 748 were confirmed to have focal brain lesions and epilepsy according to the current International League Against Epilepsy (ILAE) definitions.18 To be able to address generalizability of the findings across lesion etiologies, we selected the two most common etiologies in our dataset into this study [astrocytoma (n=201) and ischemic stroke (n=134)].
Stroke sample
The inclusion criteria were: (i) late seizures consistent with symptomatic epilepsy caused by an ischemic stroke, (ii) one or more ischemic focal stroke lesions in the MRI, (iii) no other brain lesions or structural abnormalities, (iv) no history of epileptic seizures prior to the stroke. Cases with diagnostic uncertainty whether they had focal-onset epilepsy or unclear etiology of epilepsy were excluded. In total, 76 patients fulfilled all criteria and were included in the study (Figure 1).
Tumor sample
The inclusion criteria were: (i) symptomatic epilepsy caused by an astrocytoma, (ii) one or more tumors with identifiable borders in the MRI, (iii) no other brain lesions or structural abnormalities, (iv) no history of epileptic seizures prior to the astrocytoma diagnosis. Cases with diagnostic uncertainty whether they had focal-onset epilepsy, unclear etiology of epilepsy or evidence of metastases were excluded. In total, 94 patients fulfilled the inclusion criteria (Figure 1).
Clinical phenotyping
Demographical and clinical data from the selected patients were obtained based on a detailed review of the medical records. Lesion etiology, gender, age at diagnosis, age at first seizure, age at occurrence of the lesion, seizure semiology, EEG findings, number of antiepileptic drugs (AEDs), presence of seizures at best AED therapy (in tumors before any surgery) and status epilepticus episodes were retrieved from the clinical records. In addition, tumor grade (I-IV) was extracted for patients with astrocytomas.
Time of occurrence of the lesion was defined as the time of first symptoms for stroke in the stroke sample and first brain scan showing the lesion in the tumor sample. The epilepsy types were classified to focal only (focal aware or focal impaired awareness), and FTBTC in accordance with the current ILAE classification.18,19
Lesions
Brain lesions were manually drawn on the high-resolution MR images in the subjects’ native space using FSLeyes software [version 0.31.2, (Jenkinson et al., 2012)]. All slices in the T1 or T2w sequence were examined, and the lesion was approximated on each slice in all three planes. Non-lesioned voxels were assigned value 0 and lesioned voxels value 1, producing a three-dimensional binary lesion mask. The individual MRI was then transformed to the MNI152 standard space using FMRIB’s linear image registration tool (FLIRT) implemented in FSL.20-22 Nonlinear registration was not used because structural lesions may affect the gross brain anatomy, leading to bias when using other commonly used nonlinear transformations. Nearest-neighbor interpolation was used to preserve dichotomous voxel classification (lesioned vs not lesioned voxels). The manually drawn lesion mask was registered to MNI152 standard space using the registration matrix obtained from the transformation of the anatomical image to MNI space. The alignment of the transformed images and resulting localization of the lesion in MNI space were manually inspected and edited to ensure accurate anatomical localization of the lesion. Lesion masks were used in both the region-of-interest analyses and voxel-wise analyses.
Regions-of-interest
A cerebral cortex mask was defined by combining all cortical regions in the Harvard-Oxford Cortical Structural Atlas with 0.25 threshold.23 Cortical involvement was evaluated by quantifying the number of lesion voxels intersecting with the cerebral cortex mask and dividing the number of cortical voxels with the total lesion volume. Lesions with at least half of the voxels located in the cerebral cortex were defined as predominantly cortical lesions. Lesion laterality was evaluated visually using the lesion masks. With bilaterally extending lesions, the hemisphere with the majority of voxels was defined as the predominant side of the lesion.
Lobar regions-of-interest (ROIs) were defined in the right and left hemisphere separately to the frontal, parietal, occipital and temporal lobe using the Harvard-Oxford Cortical Structural Atlas by combining all cortical subregions within each lobe. Lesioned voxels in each ROI were calculated and predominantly involved lobe was defined as the lobe with highest number of lesioned voxels.
Statistical analyses
Differences in demographical and clinical characteristics between samples and epilepsy types were compared using independent samples t-test or Mann-Whitney U-test (continuous variables) and Chi-Square or Fisher Exact test (categorical variables), as appropriate.
Differences in lesion location (laterality and cortical involvement) between cases with focal vs FTBTC seizures in the whole sample were investigated using Chi-Square or Fisher Exact test, as appropriate. In addition, the degree of cortical involvement (percentage of the voxels located in the cerebral cortex) was compared between the groups using independent samples t-test. Finally, a binary logistic regression analysis with seizure type as the dependent variable, and predominant side, cortical involvement and lesion type as independent variables was conducted to study if these factors were independently associated with seizure generalization.
To localize the findings within the hemisphere at lobar-level, ROI analyses were conducted by comparing the number of voxels in each lobe between patients with focal only and FTBTC seizures. The significance of the difference was investigated using Mann-Whitney U tests because the highly skewed distribution of the numbers of voxels in each lobe. Bonferroni correction was used to control for increased type I error due to four lobes included in the analysis. The other hemisphere was used as a negative control to verify the hemispheric specificity of the findings. Independence of the total lesion size was investigated by repeating the analysis with percentages of voxels in an ROI of total lesion voxel, and independence of etiology by repeating the analysis in strokes and tumors separately. Finally, by comparing the proportions of patients with FTBTC seizures in patients with lesions predominantly involving the identified lobe vs. other brain regions using Fisher’s Exact test, and the clinical relevance of the significant findings was quantified by calculating odds ratio for FTBTC seizures for the identified lobe compared to all other lesions using binary logistic regression controlling for lesion type.
Voxel-based lesion symptom mapping
Voxelwise lesion locations in patients with focal vs FTBTC seizures were compared using voxel-based lesion symptom mapping (VLSM) in both datasets combined. VLSM was conducted using NiiStat software (https://github.com.neurolabusc/NiiStat) according to the published recommendations.24 Lesion etiology and lesion size were used as nuisance covariates. The analyses were conducted across the whole brain in voxels affected in at least 5% (n≥9) of the cases and confirmed with thresholds of at least 10% (n≥17) and n≥1. Statistical significance was set at P<0.05 corrected for family-wise error (FWE) using Freedman-Lane permutation with 2000 permutations. The main analysis was repeated in strokes and tumors separately to identify any etiology-dependent effects.
Ethical statement
The study protocol was approved by Turku University Hospital Clinical Research Services Board, and the study was conducted according to the principles of the Declaration of Helsinki. Due to the retrospective register-based nature of the clinical data collection and large sample size, the need for separate ethical board review and obtaining written consent from the patients was waived.
Data availability
Voxelwise maps are available upon reasonable request. The original data cannot be made publicly available.
Results
Demographics and lesions
The demographical and clinical data of the lesion samples are presented in Table 1. There were no differences in the proportion of patients with FTBTC seizures between etiologies (Table 1). In patients with focal seizures only, seizures with impaired awareness were more common in strokes compared to tumors (Table 1). Focal slowing in EEG was more common in strokes compared to tumors (Table 1). Stroke patients were older and had a longer delay between the discovery of the lesion and onset of seizures compared to tumor patients (Table 1).
Table 1. Demographical and clinical data
Lesion locations were heterogeneously distributed across the brain (Figure 2A) with tumors preferentially localizing to the basal ganglia, insula, temporal lobes and frontal lobes (Figure 2B), and strokes to the regions supplied by the middle cerebral artery (Figure 2C).
Figure 2. Lesion overlap
Lesion overlap in A) the whole sample (n=170), B) tumor sample (n=94) and C) stroke sample (n=76). The maximum overlap of lesions at any voxel was 18.8%, 28.7% and 23.6% of the sample, respectively.
Patients with FTBTC seizures were slightly younger compared to patients with focal seizures only but there were no other significant differences between the groups in demographic or clinical characteristics (Table 2). Demographic data according to the seizure type for the stroke and tumor samples separately are available in the supplementary material (Table S1 and S2, respectively).
Table 2. Demographic and clinical data according to the seizure type
Regions-of-interest analyses
FTBTC seizures were more common in patients with lesions predominantly localized to the cerebral cortex compared to subcortical lesions (65.3% vs 44.2%, p = 0.01, Figure 3A), and the right hemisphere compared to the left (68.5% vs 50.6%, p = 0.02, Figure 3B). The mean (SD) proportion of voxels of the lesion in the cerebral cortex was 62.0% (19.8) in lesions associated with FTBTC seizures and 54.9% (22.7) in lesions associated with focal seizures only (p = 0.03). The laterality effect remained significant when excluding 21 patients with bilateral lesions from the analysis (68.3% vs 51.4%, p = 0.04). In the logistic regression analysis, both predominantly cortical (OR 2.50, 95% C.I. 1.21-5.15, p = 0.01) and right-sided lesions (OR 2.22, 95% C.I. 1.17-4.20, p = 0.01) were independently associated with FTBTC seizures, while lesion etiology was not (p = 1.0). There were no significant interaction effects between predominantly cortical lesion location, lesion laterality and cortical involvement. Adding age at lesion discovery to the regression model did not change the significance of the effects. There were also no differences in the proportion of predominantly cortical (79.6% vs 69.7%, p = 0.14) or right-sided lesions 51.1% vs 53.9%, p = 0.71) between tumors and strokes.
Figure 3. Lesion locations associated with focal to bilateral tonic-clonic (FTBTC) seizures
Proportion of patients with FTBTC seizures according to the predominant side of the lesion (A), predominantly cortical / subcortical lesion location, and involvement of the right frontal lobe (C). Voxel-lesion symptom mapping (VLSM) z map showing brain regions associated with FTBTC seizures (red-yellow scale) and focal seizures (blue-green scale) (D). The sagittal section is from the right hemisphere. There were no voxels significantly associated with the seizure type when corrected for multiple comparisons in the whole search volume.
In the lobar ROI analyses, lesions associated with FTBTC seizures involved the right frontal cortex more than lesions associated with focal seizures only [median (IQR) voxels: 781 (4-3386) vs. 416 (0 – 1929), p = 0.007, corrected p = 0.03). There were no significant differences between the seizure types in other lobes in the right or in any of the lobes in the left hemisphere. The involvement of the right frontal lobe was also significant when accounting for lesion size (p=0.006), and when analyzing strokes (p=0.048) and tumors (p=0.03) separately]. Lesions predominantly located in the right frontal cortex were associated with FTBTC seizures (OR 4.41, 95% C.I. 1.44-13.5, p = 0.009) compared to lesions located in other regions independent of lesion etiology (p = 0.96) (Figure 3C). There also was no significant interaction effect between lesion type and location in the right frontal cortex (p = 0.46).
Voxel-based lesion symptom mapping
The results from the voxelwise VLSM analyses showed clear right-laterization of lesion locations associated with FTBTC seizures (Figure 3D, Figure S1). However, the VLSM analysis did not identify any single voxels significantly associated with FTBTC seizures (Figure 3D).
Discussion
The main finding of the present study is that lesion location is associated with secondary generalization of focal seizures (FTBTC seizures). Specifically, cortical lesions, lesions in the right hemisphere and right frontal lobe are associated with an increased risk for FTBTC seizures. These effects were independent of lesion etiology. However, lesion associated with FTBTC seizures did not localize to any single anatomical region within the right frontal cortex, which is in agreement with the current views that seizure generalization is a multifactorial process involving widespread brain regions rather than localized to a single hub in the brain.11,12
Previous lesion mapping studies in epilepsy have mainly focused on identification of clinical risk factors for epilepsy and crude anatomical localization of brain regions associated with seizures and epilepsy.5,7,25 Across etiologies, there is consensus that lesions in the cerebral cortex are associated with a higher risk for epileptic seizures compared to subcortical lesions, e.g. basal ganglia and cerebellum.4,5,7,8 In stroke, lesions in the anterior circulation are associated with increased late seizure risk but result of more detailed localization into which lobes are associated with an increased risk for late seizure have been negative or inconsistent between studies.26-30 Studies investigating seizure risk in brain tumors have linked increased seizure risk to varying brain regions, including the medial temporal and frontal lobes.6,8-10 However, there doesn’t seem to be consistent evidence on which brain regions are most susceptible for the development of lesion-induced epileptic seizures across etiologies.
The present study focused on identifying lesion locations associated with secondary seizure generalization rather than epileptic seizures per se. Our results show that cortical lesions increase the risk for FTBTC seizures, adding to the current knowledge that cortical lesions are more prone to cause any type of seizures.5,7,25 Cortical lesions could contribute to the risk of seizure generalization in multiple ways. Greater lesion burden in the cerebral cortex may increase the amount of brain tissue with reduced seizure threshold, causing more widespread or multifocal discharges, or making it easier for epileptic activity to spread from cortical region to another, eventually leading to secondary generalization to FTBTC seizures.12,31 Cortical lesions may also lead to a disconnection and loss of cortical control over spontaneous oscillations of subcortical hubs, such as the thalamus, which is known to generate synchronous abnormal neuronal activity in generalized seizures.11,12,32,33
Lesion location in the right hemisphere was another independent risk factor for FTBTC seizures in the present study. While previously reported in the literature, the reason for increased risk for secondary generalization of seizures with right hemisphere lesions is not clear. Our finding is in agreement with earlier evidence of right-sided stroke lesions associated with an increased risk for late seizures.30 It has been suggested that the left hemisphere may have lower seizure threshold compared to the right hemisphere.34 While we can only speculate on this, seizure generalization to the contralateral hemisphere may occur more easily in patients with lesion induced epilepsy originating from the, “less-epileptogenic”, right sided hemisphere. Seizure generalization from the left hemisphere to the right hemisphere may be less frequent, due to the higher seizure threshold for the right hemisphere. Our findings also indicate that lesion location in the right frontal cortex may carry a particularly high risk for secondary generalization. However, similarly to many other studies6,9,26-30,35, we did not identify any single common hub that, when lesioned, would be significantly associated with epileptic seizures. There are only very few previous studies analyzing the lesion location in a voxel-wise manner and comparing patients with FTBTC to focal seizures. The results from these studies indicate that gliomas involving the corpus callosum13 or premotor cortex8 could increase risk for FTBTC seizures, but these findings have not been replicated or confirmed in other studies including the present study.
Patients with FTBTC seizures were slightly younger compared to patients with focal seizures only. This is in line with previous observations that younger age is associated with increased risk for late-onset seizures5 and could be explained by slow neuroplastic changes, which are known to be more pronounced in younger individuals.36 However, age was not independently associated with seizure generalization in the present study and adding age to the regression model did not change the significance of the lesion laterality or cortical location effects. The strengths of the present study include a focus on seizure generalization and detailed lesion localization by only including patients with high-quality, modern brain MRI with a clearly identifiable focal lesions without mass effects. The analyses were conducted by using several approaches, including a region of interest analyses of crude lesion localization (cortical-subcortical, right-left hemisphere, lobes) and a whole brain voxelwise analysis to identify specific brain regions. Our study was also not restricted to single lesion etiology but included two clinically different lesion types to identify brain regions, which could be important for secondary generalization across etiologies.
There are however some limitations that should be considered when interpreting the results of the present study. First, although our findings indicate that lesion-based localization of FTBTC seizures are independent on lesion etiology, our analyses were limited two lesion types (ischemic strokes, astrocytomas). To what extent these findings generalize to other etiologies, remains to be investigated in subsequent studies. Second, although all of our cases were diagnosed at a university hospital and carefully re-evaluated by the investigators, due to the retrospective nature of the study, it is possible that the clinical characterization of the epilepsy is incorrect in some patients. For example, patients may not report FTBTC seizures because of fearing losing a job or driver’s license. However, incorrect characterization of seizures would lead to increased noise in the data and bias us against the present findings indicating that lesion location is associated with FTBTC seizures. Finally, it is possible that our sample size may not have been sufficient to identify associations between lesions to individual brain regions and seizure generalization. However, if present, such weak associations are likely to have only limited significance in clinical practice.
In conclusion, brain lesions localizing to the cerebral cortex and the right hemisphere are associated with increased risk for secondary seizure generalization in patients with lesion-induced epilepsy. Our findings further suggest that lesions in the right frontal cortex may be particularly susceptible for development of FTBTC seizures. These findings can help to better identify patients at risk for FTBTC seizures.
Data Availability
Voxelwise maps are available upon reasonable request. The original data cannot be made publicly available.
Conflicts of interest
The authors report no relevant conflicts of interest.
Financial disclosure
JN, MB and LN have nothing to report. JJ has received research funding from the Finnish Foundation for Alcohol Studies, Finnish Medical Foundation, Finnish Parkinson Foundation, University of Turku and Turku University Hospital (VTR funding), and lecturer honorarium from Lundbeck.
90,000 types of disorders and possible treatment
Regardless of what caused the brain damage, they all entail the manifestation of neurological dysfunctions in relation to hearing, speech, vision or movement. Elimination of these disorders requires a complex of rehabilitation measures, which, alas, is almost impossible to pass at home or in a non-specialized clinical hospital, or it will not give a positive effect. Why this is so, we will try to explain in this article.
Various brain lesions due to stroke, traumatic brain injury or other diseases lead most often to neurological disorders: motor, speech, cognitive and others.
Depending on the severity of the disease and the location of the damage in the brain, the intensity and reversibility of these neurological disorders can be different. In any case, after receiving emergency medical care and treating the underlying disease, a period of rehabilitation begins, designed to restore the lost functions or, if this turns out to be impossible for medical reasons, to teach a person to live a full life after the transferred diseases.
In the scientific medical community, most neurologists and other specialists agree that the period of active rehabilitation of victims of brain lesions should begin in the first two to three weeks after the acute phase of the disease. And the greatest effectiveness of rehabilitation treatment falls on the first three to six months, in the next six months the gains are consolidated. In fact, depending on the nature and severity of the consequences, rehabilitation can last up to two years – often this is how long it takes to restore speech.
Rehabilitation of movement disorders
Here we will focus on warnings against common mistakes that people make when they decide to undergo home rehabilitation after injuries and other brain diseases that have caused movement disorders.
- As already mentioned, early rehabilitation after brain damage is indicated, but many patients and their relatives take this too literally. Indeed, usually, for example, after ischemic strokes, motor rehabilitation begins in a week (if there are no contraindications), after hemorrhagic strokes – after two to three weeks.Therefore, it is impossible to start it until there is confidence in its timeliness with the attending physician, with whom you agree on the volume and time of the beginning of rehabilitation measures.
- Choose your doctor carefully, because in Russia very many of them tend to prescribe treatment according to the standard prescribed scheme, performing a certain “ritual”, without taking into account the individual characteristics of the organism. So, many began treatment after the words: “The massage has not harmed anyone yet,” and then they discovered spasticity and further deterioration of motor functions.
- Continuing the topic of treatment “according to the scheme”, let’s say about the prevention of contractures, prescribing one or two times a day to engage in treatment with a position lasting up to one and a half to two hours. However, if the rest of the twenty-two hours the arms and legs are in the wrong positions, then the benefit from this two-hour procedure will be zero.
- Intense and prolonged passive limb development can be not only useless, but also dangerous. In addition to the fact that it is not so much the victim who trains as the person giving him the massage, the intensified active massaging movements create the risk of dislocation and loosening of the joints – with paresis, and the risk of microtrauma and paraarticular ossification – with spastic paralysis.Rotational massage of the joints of the legs, which is carried out by a non-specialist, is extremely dangerous.
- Ideomotor gymnastics, or the representation of one’s own movement without the movement itself, can be not only useless, but also harmful: if the patient only “dreams” of movement, without making any internal efforts for this, volitional muscle reconstruction of the imaginary movement does not occur , forming the so-called central sprouting.
- In view of the fact that in cases with spastic paralysis the presence of muscle atrophy is not observed, active or power load on the intact arms or legs will lead to “robbing” the affected side, and in spastic muscles – to increased spasticity.So, after strokes, training the quadriceps muscles of the thigh and biceps is strictly contraindicated.
- When prescribing a massage, it is not enough to indicate the area of massage, but it is also necessary to recommend its nature, type of impact and, of course, set a task. So, the types of massage include: acupressure, structuring, resorption, soothing, hydrodynamic and others. And the tasks can be, for example, the following: mobilization of muscles and tendons, elimination of trigger zones, or mild edema, and others.
- Training on simulators involves not only carrying out certain exercises, but also performing them correctly. The person being rehabilitated can adapt to perform certain exercises using the healthy side or strong muscles. For example, when exercising on stationary bicycles, the exercises may be performed by the patient with only one leg, or using only the extensor or flexor muscles, and from the outside it may seem that the exercise is being performed correctly while it is harmful.
- Underestimating the patient’s mental and psychological state is another typical mistake people make when trying to restore motor functions. But the overwhelming majority of patients after brain injuries and serious illnesses are immersed in depression, in which they remain for several years, if they are not provided with the proper psychological assistance. Depression takes away from the affected people the moral strength to engage in therapeutic exercises, for the motivated and volitional participation of patients in their rehabilitation treatment.
- Ignorance or underestimation of the importance of an integrated approach in motor rehabilitation, which implies a rational combination of various methods of restorative medicine: where drug treatment is only a background for physiotherapy, followed by prevention of contractures, massage, psychotraining, and so on.
- And finally, the brain is in close “contact” with all parts and organs of a person, and any actions in the brain send them certain “commands”, and vice versa, any processes in the body are reflected in the activity of the corresponding areas of the brain.That is why movement exercises train not only muscles and tendons, and are the prevention of concomitant diseases, but also contribute to the speedy regeneration of nerve connections.
The above information is provided in this section in order to understand the importance of professionalism in assisting in the recovery of people who have suffered brain injury, strokes or other serious illnesses resulting in motor dysfunctions. At the forefront of any medical appointment should be a task or a set of interrelated tasks, to the solution of which various methods of restorative treatment are directed, and aimless or “chaotic” treatment at home not only will not lead to the desired results, but will also shift the vector of recovery in the other direction.
Restoration of speech dysfunctions
Organic lesions of the speech areas of the cerebral cortex can be the result of a tumor, stroke, trauma or inflammation. They lead to a systemic speech disorder called aphasia.
In total, there are about eight types of aphasia, which can be conditionally divided into two groups: sensory aphasia and motor aphasia. The first group is characterized by the fact that a person ceases to understand speech, but is able to pronounce words and phrases.Motor aphasia is manifested in the fact that a person hears and understands speech, but cannot pronounce words and phrases. Speech problems occur in a quarter of people who have had a stroke.
Speech dysfunctions entail a whole complex of negative mental states in people who have suffered organic lesions of the cerebral cortex, such as tearfulness, irritability, aggression, inadequate assessment of reality and depression.
Therefore, when restoring speech functions, several specialists are involved, these can be speech therapists, neuropsychologists, teachers, psychiatrists and psychologists.Recovery of speech takes a long time, much more than it takes for motor rehabilitation – two years, and in some cases, five to six years.
It is important to remember that often people with organic brain lesions do not understand that they have impaired counting, writing or speech, and if they do, then they believe that over time everything will work out by itself. In such cases, it is important to explain the situation to them, set a task for them and convince them of the achievability of the goal. You should also show the connection between the efforts made and the successful result, tell that the desire to achieve the goal and optimism will do more on the way of recovery than any drug therapy.
A typical mistake in speech rehabilitation with non-specialists is the wrong choice of exercises and training regime. This will not directly harm the victim, but it will deprive him of faith in success after many unsuccessful activities. This is why it is so important to seek professional help.
To work on restoring the patient’s speech requires fantastic patience, it is very important not to get annoyed while studying with him and constantly keep the rehabilitated person interested in them. As a rule, daily leisurely calm conversations are recommended, as well as singing melodious songs, reciting simple children’s poems, and reading an ABC book.
If you nevertheless decide to deal with the victim on your own, be sure to buy literature, find out from a speech therapist and other specialists about existing techniques, use them in a comprehensive manner and in accordance with the existing instructions of specialists.
Correction of cognitive impairments
Most often, cognitive disorders are caused by ischemic strokes occurring against the background of diabetes, arterial hypertension and atrial fibrillation.
In the last decade, the results of many studies have appeared that convincingly prove that cognitive disorders negatively affect the entire process of rehabilitation, the restoration of all impaired functions: motor, visual, speech and others.Undoubtedly, other factors also affect the rehabilitation process:
- Age,
- Size of the focus of organic brain damage,
- Localization of injuries,
- Severity of the disease
- Time of initiation of treatment and rehabilitation,
- Presence of concomitant diseases.
But previously, cognitive impairment was not considered an aggravating factor in rehabilitation.
The rates of rehabilitation treatment are significantly influenced by such affective states as emotional lability, apathy, mania, anxiety and depression.Moreover, it was previously believed that depression is only a reaction to a past illness, although now it is known that, firstly, in frequency it exceeds all other mental disorders, and secondly, it is also formed due to personal, social and other factors.
For this reason, in the rehabilitation treatment of patients who have received organic brain lesions, it is important to involve various specialists from the field of medicine, pedagogy, psychology and other fields of knowledge.An integrated approach to rehabilitation, a rational combination and rotation in the application of many different methods, becomes obvious. Since today the fact of the interconnection of all possible neurological disorders after the resulting brain lesions can be considered proven, we inevitably come to the conclusion how unsuccessful can be rehabilitation at home and even in an ordinary clinical hospital. After all, comprehensive rehabilitation is not just a set of various restorative techniques – it is an individual approach, agreement and approval by the community of doctors of a whole range of rehabilitation measures and further multistage targeted restorative treatment.
Center news – MEDEXPERT
10.07.2019
MRI for epilepsy
The main diagnostic method for epilepsy is considered to be a neurophysiological study, primarily EEG – an electroencephalogram. Often, in combination with an EEG, MRI is also prescribed – magnetic resonance imaging. Despite the fact that this method is clarifying and is not used for the primary diagnosis of epilepsy, it can provide important additional information that is difficult and sometimes impossible to obtain with the help of other examinations.
In particular, MRI in epilepsy can detect neoplasms, vascular anomalies, foci of multiple sclerosis, purulent inflammation, congenital anomalies of the brain structure. MRI with high accuracy reveals inflammatory processes, traumatic brain lesions, vascular malformations, sclerotic disorders of the hippocampus. One of the main advantages of MRI is that it helps to see even the smallest structural changes in the cerebral cortex. Before surgery for epilepsy, MRI is used to clarify the boundaries of surgery.If it is necessary to establish the benign or malignant nature of the tumor in epilepsy, contrast-enhanced MRI is used. The power of the tomograph for all types of examination for epilepsy must be at least 1.5 Tesla. As a rule, this power is not only necessary, but also sufficient.
The definitive indication for MRI is the first unprovoked epileptic seizure. In this case, without an MRI scan, the diagnosis cannot be considered confirmed. In addition, MRI is prescribed when drug treatment is ineffective in patients diagnosed with primary generalized epilepsy, as well as in recurrent epileptic seizures and paroxysmal states of unknown origin.
Magnetic resonance imaging is a completely safe examination method. However, it is considered undesirable in children under 3 years of age with a single epileptic seizure. In this case, MRI is performed when the child reaches 3 years of age. In addition, MRI is considered inappropriate for epilepsy associated with traumatic brain injury or meningoencephalitis, if seizures do not recur after an acute period.
Vascular diseases of the brain
Vascular diseases account for more than 40% of all pathology of the nervous system; this is one of the most common diseases in neurology.Everyone is well aware of such formidable diseases as strokes and heart attacks, but these are only complications of long-term progressive vascular diseases of the brain. The most common causes of the development of progressive discirculatory encephalopathy is a combination of cerebral artery atherosclerosis and hypertension. Other possible causes are vasculitis in systemic inflammatory diseases, congenital anomalies in the structure of the heart, blood vessels, spine, venous disorders, pathology of the vertebral arteries, hereditary blood diseases, constitutional features of the autonomic nervous system with arterial hypotension, and rarely other causes.Cerebrovascular insufficiency develops in stages.
At the first stage of discirculatory encephalopathy, subjective unstable complaints of headaches, impaired performance, sometimes swaying and other mild nonspecific symptoms in the form of irritability, sleep disturbances predominate.
At the 2nd stage , the symptoms become more pronounced and prolonged, objective symptoms in the form of crises join, more often against the background of an unstable increase in blood pressure, impaired coordination, changes in reflexes and other symptoms.
At the 3rd stage there can be persistent organic manifestations – memory impairment, impaired coordination, transient cerebral circulation disorders, complications in the form of strokes and their consequences are possible.
Diagnostics
Diagnosis of cerebrovascular insufficiency is carried out by a neurologist. Methods such as collection of anamnesis, assessment of cardiac hemodynamics – blood pressure, pulse and other indicators are used, cognitive (mental) activity is checked both during a conversation with a patient, and with the help of specially designed questionnaires and test tasks with a quantitative assessment of the points scored.
Laboratory tests – the content of cholesterol and its fractions, blood glucose levels and other biochemical parameters help in assessing the general condition.
An important method for assessing cerebral circulation is Doppler ultrasound with color Doppler mapping of the vessels of the neck and brain. This method allows you to identify atherosclerotic changes in the arteries and assess the blood flow in all major arteries of the brain.
In modern conditions, it is also necessary to visualize the brain using magnetic resonance imaging or computed tomography with angiography of the cerebral arteries, which will make it possible to assess the structural changes in the brain, to identify the consequences of stroke.
At a late stage of the disease, multifocal vascular lesion of the brain is diagnosed on magnetic resonance imaging in the form of zones of atrophy and leukoaraiosis, which clinically, as a rule, manifests itself as a decrease in memory and impaired cognitive functions.
Treatment
Methods of treatment of cerebrovascular insufficiency are various, you just need to remember that vascular accidents are easier to prevent. Taking vascular, lipid-lowering, antihypertensive and metabolic therapy should be persistent and long-term.
The most important role belongs to lifestyle modification – this is smoking cessation, rational sports activities, diet and sleep.
Be healthy! 90,000 causes of occurrence, under what diseases occurs, diagnostics and methods of treatment
IMPORTANT!
The information in this section cannot be used for self-diagnosis and self-medication. In case of pain or other exacerbation of the disease, only the attending physician should prescribe diagnostic tests.For a diagnosis and correct treatment, you should contact your doctor.
Stuttering: causes of occurrence, for what diseases it occurs, diagnostics and methods of treatment.
Definition
Oral speech is a complex multicomponent process in which not only the structures of the maxillofacial apparatus are involved, but also the centers of the brain located in the cerebral cortex. It is in these structures that, in most cases, certain pathological processes leading to the development of stuttering are localized.
Stuttering (stammering) is understood as a feature of speech, which is characterized by frequent repetition or prolongation of sounds, syllables or even words in combination with frequent pauses, which disrupts the fluency of oral speech.
Today there are different figures characterizing the number of stuttering people – from 1 to 5%. However, all experts are unanimous that stuttering occurs 4 times more often among males than among females, and also that in 90-95% of cases, stuttering occurs at the age of 2 to 7 years.
This speech defect often leads to communication problems, which consist not only in the difficulty of perceiving such speech from the side of the listener, but also associated with the internal emotional experiences of the stuttering person.
Types of stuttering
There are the following types of stuttering:
- Neurotic stuttering, the development of which is usually based on some kind of traumatic factor. This type of stuttering is psychogenic and is not based on structural damage to the brain or peripheral parts of the speech apparatus.
- Neurosis-like stuttering – unlike neurotic, it develops against the background of organic damage to the nervous system.
- Mixed stuttering – develops against the background of both a psychogenic factor and a true lesion of the nervous system.
Possible causes of stuttering
Stuttering is based on various spasms of the muscles of the speech apparatus. They develop due to abnormal impulses emanating from the motor speech centers of the brain.The cause of the pathological work of these parts of the central nervous system can be both structural and functional disorders. The latter are characterized by the presence of an excitation focus in some part of the brain, which activates the motor speech center and leads to the development of convulsive readiness of the muscles involved in articulation and voice formation.
In the case of the development of tonic seizures, a prolonged muscle spasm occurs, resulting in a delay in speech. Unlike tonic seizures, clonic seizures are a series of short-term seizures that lead to involuntary repetition of sounds or syllables.
Both the one and the other type of seizures lead to impaired fluency of speech and stuttering.
Diseases causing stuttering
Among the factors influencing the development of stuttering, doctors distinguish the following diseases and conditions:
- emotional lability and dysfunction of the autonomic nervous system;
- mental trauma;
- traumatic brain injury;
- infectious brain damage;
- perinatal damage to the central nervous system of a child, usually associated with a violation of oxygen supply to the structures of the brain during the prenatal period of development, during childbirth and in the first hours and days of life.
90,019 stroke;
Which doctor should i contact for stuttering
Traditionally, the initial examination of a patient with stuttering is carried out
a neurologist. After a detailed questioning of the patient, the purpose of which is to clarify the circumstances of the appearance of stuttering, possible provoking factors, the doctor specifically examines the nervous system for other signs of neurological abnormalities. In the treatment of stuttering, doctors resort to the help of a speech therapist, a psychologist. Often, for the treatment of stuttering, especially in the absence of organic damage to the nervous system, the neurologist sends the patient to a psychiatrist’s consultation in order to identify some mental abnormalities leading to the development of stuttering.
Diagnostics and examinations for stuttering
The diagnosis of stuttering begins with a neurological examination, during which the neurologist may suspect the presence of structural damage to the brain or, conversely, receive no evidence of the presence of organic damage. In this case, the doctor resorts to methods of additional laboratory and instrumental diagnostics in order to confirm or exclude various organic lesions. EEG and neuroimaging methods come to the fore.
Electroencephalography (EEG) is one of the essential methods of additional examination of a patient suffering from stuttering. EEG helps to differentiate neurotic and neurosis-like stuttering, revealing signs of seizure activity in certain parts of the brain.
Epilepsy and epileptic syndromes
Epilepsy is a chronic neurological disease characterized by the sudden onset of seizures caused by excessive excitation of neurons in the cerebral cortex.
Symptoms and manifestations of epilepsy
Epileptic seizures are very diverse. Basically, these are tonic (prolonged contractions of a particular muscle group) and clonic (rapid, rhythmic contractions of all skeletal muscles) convulsions, change or loss of consciousness, respiratory failure. The duration of the seizures is 3-4 minutes on average. Often, the onset of an attack is accompanied by an aura – a combination of sensations and experiences that the patient experiences before the attack. The aura manifests itself in different ways, depending on the localization of the focus of excitation in the brain.Most often these are visual (flashes, spots, stripes), auditory (sounds, noises, words, music), tactile (numbness, tingling, chills) hallucinations, as well as mental manifestations (déjà vu, feelings of fear and horror).
A change in the electrical activity of nerve cells or a violation of the processes of excitation and inhibition of the cerebral cortex can occur due to a number of diseases or brain injuries: tumor, hydrocephalus, encephalitis, hypoxia, traumatic brain injury, vascular pathology, metabolic disorders.In this case, these seizures are called epileptic syndrome or symptomatic epilepsy. Therefore, when seizures appear, it is necessary to check the child for all possible diseases so that the therapy of seizures is aimed, first of all, at eliminating the cause. The main thing, when symptoms of eplepsia appear, is a deep diagnosis and determination of the pathogenesis of the disorder.
Most often, children experience fibril convulsions, which appear against a background of high temperature. However, if the doctor fails to find somatic pathologies that cause repeated, regular seizures, then epilepsy is diagnosed.More than 40 forms of epilepsy are known with different age of the onset of the disease, clinical presentation and prognosis. 75% of epilepsies have a good therapeutic prognosis and disappear by the age of 14.
Diagnostics and treatment
The diagnosis and treatment of epilepsy and epileptic syndrome should be started after the first seizure that appears, since in childhood, cognitive impairment may appear even after one seizure. One attack can lead to subsequent attacks, since excitement from the focus of pathology can spread through neural networks to other areas.Prolonged and frequent seizures can cause neuronal death and lead to the development of negative consequences: trauma during seizures, emotional instability, impaired personality formation, delayed psychomotor development, and the development of mental retardation. It is possible to change the personality according to the epileptoid type: dysphoria, irritability, spiteful melancholy mood.
The diagnosis of epilepsy or epileptic syndrome is made by a pediatric neurologist or epileptologist. First of all, the doctor asks parents about the frequency, duration, time of onset of seizures, and the presence of an aura.Further, it is necessary to instrumentally assess the electrophysiological activity of the brain, to identify the localization of areas of increased excitability using EEG. It is also used EEG with light or audio stimulation, long-term (night) EEG monitoring. CT and MRI of the brain can check structural changes in the brain. Additionally, a biochemical blood test and a lumbar puncture are prescribed. The treatment of epilepsy and epileptic syndrome is based on pharmacotherapy, individually selected by the doctor, depending on the type of seizures and the form of the disease.There is a wide range of anticonvulsants that can help control seizures and significantly reduce the number and duration of seizures.
Curative pedagogy
If a child has signs of a delay in psycho-speech development, then it is necessary to conduct classes according to the program of curative pedagogy with a defectologist, speech therapist-defectologist. A psychologist will help the child to learn self-control to cope with psycho-emotional and personal disorders.In cases of complex combined disorders, the Neurorehabilitation program has proven itself well, allowing several doctors and correctional specialists to work on the patient’s recovery. The interdisciplinary approach used in neurorehabilitation allows to achieve the most coordinated actions of doctors.
REVERSIBLE AND UNREVERSIBLE CHANGES OF THE BONE-JOINT SYSTEM IN TYPE I Gauche disease | Solovyova
Introduction
Gaucher’s disease (GD) is the most common form of hereditary fermentopathies combined into the group of lysosomal storage diseases.The disease is based on a hereditary deficiency in the activity of acidic β-glucosidase (glucocerebrosidase, HCB), a lysosomal enzyme involved in the degradation of cellular metabolic products [1-3]. The disease occurs with a frequency of 1: 40,000 to 1: 60,000 in all ethnic groups; in the population of Ashkenazi Jews, the incidence of the disease reaches 1: 450-1: 1000 [4].
BG was first described in 1882 by the French physician Philippe C.E. Gaucher, who isolated cells pathognomonic for this disease – macrophages that accumulate lipids, later called Gaucher cells.In 1901, HD was classified as a lysosomal storage disease with an autosomal recessive mode of inheritance. In 1965, it was found that the cause of the disease is a congenital deficiency of HCB, a lysosomal enzyme involved in the cleavage of glycosphingolipids in the membranes of destructing cells, in particular glucocerebroside [3, 4].
BG is inherited by an autosomal recessive mechanism. The presence of two mutant alleles of the HCB gene is associated with a decrease (or absence) of the catalytic activity of HCB, which leads to the accumulation of unused lipids in the cytoplasm of cells [3].
HCB is found in all cells of the body, however, the deficiency of this enzyme is of greatest importance for antigen-processing macrophages, since an important function of these scavenger cells is to degrade blood cells (leukocytes, erythrocytes) that have completed their life cycle. The absence of HCB or low activity of the enzyme leads to the accumulation of unutilized lipids in the lysosomes of macrophages and the formation of characteristic accumulation cells (Gaucher cells) [3-5].
The accumulation of non-cleaved metabolic products in the cytoplasm of macrophages is accompanied by the production of pro-inflammatory cytokines by these cells, autocrine stimulation of monocytopoiesis (production of monocytes – precursor cells of macrophages – in the bone marrow) and an increase in the absolute number of macrophages in the places of their “physiological home” (spleen brain, lungs), which is manifested by hepato- and splenomegaly, infiltration of bone marrow, lungs and other organs by macrophages [5, 6].
The pathogenesis of various clinical manifestations of HD was previously associated with the accumulation of lipid-laden macrophages in organs and tissues, mechanical replacement and disruption of the normal tissue structure by Gaucher cells. According to the modern concept, the disease is based not so much on the mechanical accumulation of macrophages “overflowing with degradation products” in organs and tissues, but on the activation and disruption of numerous functions of these cells, including dysregulation of hematopoiesis and bone tissue metabolism, which presumably underlies cytopenic syndrome and damage to the skeletal system [5].
Type I BG is the most frequent clinical variant of the disease, it occurs in both children and adults. The average age of patients at the time of onset of the disease varies from 30 to 40 years. Difficulties in early detection of the disease are due to the variability of clinical manifestations in the early stages of the disease, as well as their low specificity [7]. The main symptoms of HD include splenomegaly, hepatomegaly, cytopenia, and damage to the osteoarticular system. Damage to the skeletal system in type I HD occurs in 70-100% of cases.Long bones and spine are mainly affected. At the same time, there is an exceptional heterogeneity in the nature and severity of changes in the osteoarticular system, the cause of which remains unclear to date [7, 8].
In addition, difficulties are caused by both primary and differential diagnosis of HD with a number of other hematological, oncological and somatic diseases. This is due to nonspecific clinical manifestations and similar radiation semiotics of these diseases [9].
The basis of modern diagnostics of HD is biochemical analysis of the activity of HC in blood leukocytes. The diagnosis is confirmed by laboratory tests when the enzyme activity is less than 30% of the normal value [10]. The degree of decrease in enzyme activity does not correlate with the severity of clinical manifestations and the course of the disease [3]. Modern treatment of HD is the appointment of life-long enzyme replacement therapy (PST) with recombinant HCB [5, 10]. Thus, the need for a full-fledged clinical and laboratory examination of such patients is obvious, as well as the use of a complex of methods of radiation diagnostics to assess the severity of damage to the osteoarticular system [11].
The aim of the work is to present the radiation semiotics of reversible and irreversible changes in the osteoarticular system in type I HD and to determine the role of magnetic resonance imaging (MRI) in the diagnosis and assessment of the severity of damage to the osteoarticular system in patients with type I HD.
Materials and methods
The analysis of the data of radiation studies performed in patients with HD type I who were treated in the department of orphan diseases of the Federal State Budgetary Institution of the National Medical Research Center of Hematology of the Ministry of Health of the Russian Federation from 2010 to 2018.The study included 86 patients, including 39 men and 47 women aged 25 to 84 years (median age – 37 years). In all patients, the diagnosis was confirmed by enzyme diagnostics. Examination of patients at the Federal State Budgetary Institution of the National Medical Research Center of Hematology of the Ministry of Health of the Russian Federation was carried out before the start of specific therapy.
Comprehensive radiological examination of patients with laboratory confirmed type I HD began with classical radiography. Radiography of the femurs was performed with the capture of the hip and knee joints in frontal projection.SE, T2SE, STIR. The studies were carried out on two magnetic resonance tomographs: Signa Profile from General Electric (USA) with a magnetic field induction of 0.23 T using Body Flex Coil (M and L), Ingenia from Philips (Netherlands) with a magnetic field induction of 1.5 T with dStream Anterior, dStream Posterior coils.
Figure 1 . X-ray of the femur in a direct projection. Club-shaped deformity of the distal metadiaphysis of the femur with dilatation of the medullary canals similar to Erlenmeyer flasks
Figure 1 .Plain radiograph of femurs in direct projection. Erlenmeyer flask deformity of femurs
Results
Radiography
Classical radiography was used for the initial assessment of structural changes in bones, which made it possible to establish gross pathological changes in the osteoarticular system in HD, mainly irreversible:
- Erlenmeyer flask-type bone deformity;
- bone structure heterogeneity;
- aseptic necrosis of the femoral heads;
- pathological fractures.
- Erlenmeyer flask-type bone deformity
Represents a noticeable expansion of the medullary canal of the distal femur and proximal tibia with thinning of the cortical layer from the inside due to impaired bone remodeling (Fig. 1). Erlenmeyer flask-type bone deformity was found in 97% of patients.
Figure 2. Radiographs of the femur in frontal projections. The bony structure of the distal femurs is focal sparse with alternating sclerotic components.Deformed in coarse-mesh (a) and spotty-porous types (b)
Figure 2. Plain radiographs of femurs in direct projections. Bone structure of distal femurs discharged by foci with alternation of the sclerotic component. Deformed by coarse (a) and spotty-porotic types (b)
Figure 3. X-ray of the hip joints in frontal projection. Aseptic necrosis of the head of the right femur at the stage of outcome. Secondary osteoarthritis of the right hip joint
Figure 3. Plain radiograph of hips in direct projection. Osteonecrosis of right femoral head. Secondary osteoarthrosis of right hip joint
- Bone structure heterogeneity
Bone structure in HD of varying severity was sparse due to osteopenia and general osteoporosis. The inner contours of the cortical layer became slightly blurred, the outer ones remained smooth. Mainly in the spongy ends of long bones, small focal rounded and oval limited enlightenments were detected, surrounded by reactive seals – sclerotic areas, as a result of which the bone acquired a wide-looped or maculoporous structure.Sometimes these foci of osteolysis merged into larger defects, separated from each other by crossbars, obliquely and transversely crossing the bone, and the pattern became coarse-meshed (Fig. 2). The above changes occurred in 57% of the examined patients.
- Aseptic necrosis of the femoral head
Structural changes were often identified in the femoral head and neck (Table 1). In severe cases, destructive phenomena were so pronounced that the head collapsed, which was revealed in 26% of cases.As a result, her mushroom deformity with shortening of the femoral neck was noted, which was accompanied by the development of secondary osteoarthritis of the hip joint (Fig. 3).
- Pathological fractures
Due to the thinning of the cortical layer of large tubular bones, the course of HD in 8% of cases was complicated by pathological fractures, which most often affected the proximal femur (Fig. 4).
Figure 4. Direct projection of the proximal right femur.Pathological subtrochanteric fracture of the femur with transverse displacement and partial wedging of fragments
Figure 4. Plain radiograph of proximal right femur in direct projection. Pathological subtrochanteric fracture of the femur with a transverse displacement and partial penetrations of fragments
Figure 5. MRI of the femur in coronal projection (T1-WI mode). Severe infiltration of the bone marrow of the femur and pelvic bones.Homogeneous type. Prevalence – 4 areas
Figure 5. MRI of femurs in coronal projection (Ti-WI). Expressed bone marrow infiltration of femurs and pelvic bones. Homogenous type. Prevalence – 4 areas
Table 1 shows the incidence of irreversible changes in the osteoarticular system in 86 examined HD patients.
Magnetic resonance imaging
MRI allows you to assess the state of the bone marrow in adult patients with HD, as well as to reveal:
- infiltration of the bone marrow with Gaucher cells;
- Trabecular edema, medullary and cortico-medullary osteonecrosis as manifestations of bone marrow ischemia;
- osteosclerosis;
- osteolysis.
Table 1. Bone changes (according to X-ray, n = 86)
Table 1. Bone changes (according to X-ray, n = 86)
No. | Type of changes Type of changes | Number of patients (%) Number of patients (%) |
---|---|---|
1 | Erlenmeyer flask deformity Erlenmeyer 9000 flask 2 Erlenmeyer 9000 (97) | |
2 | Heterogeneity of bone structure Heterogeneity of bone structure | 49 (57) |
3 0 necrosis of femoral heads | 22 (26) | |
4 | 9000 2 Pathological fractures Pathological fracture | 7 (8) |
- Bone marrow infiltration with Gaucher cells Specific infiltration of bone marrow with Gaucher cells had both focal and diffuse changes in the signal intensity with increased on hypointense in T1- and T2-weighted images (VI) (Fig.5). Occurred in 95% of untreated patients included in the study. The prevalence, severity and nature of bone marrow infiltration varied significantly.
MRI made it possible to carry out dynamic monitoring of the state of the bone marrow in patients over 25 years old. Against the background of PST, the structure of the bone marrow substance was restored, the percentage of fat increased, which is confirmed by an increase in the intensity of the MR signal from the bone marrow (Fig.6).
- Trabecular edema, medullary and cortico-medullary osteonecrosis as manifestations of bone marrow ischemia
On MRI, areas of trabecular bone marrow edema (reversible ischemic phase) were visualized in 27% of cases, characterized by intermediate signal intensity in T1-WI and – a new signal in T2-VI, Stir sequences (Fig. 7).
Irreversible ischemic changes in the bone marrow in patients were determined in the pineal glands (aseptic or cortico-medullary osteonecrosis), as well as in the metaphyses and diaphysis of bones (medullary osteonecrosis).MR signs of osteonecrosis varied depending on the stage of the pathological process and were found in 55% of patients. For the irreversible phase, the lesion pattern is specific, to which the hypointensive lines, which are clearly visible in T1 and T2-WI, resembling a geographic map, were attributed. Femoral heads, distal femurs, and proximal tibia were most often affected in HD (Figs. 8, 9). Cortico-medullary osteonecrosis was detected in 28% of patients, medullary – in 53%.
Figure 6. MRI of the femur in coronal projection (a, b: T1-VI mode). Bone marrow infiltration with Gauche cells before treatment (a) and its complete regression after 5 years of therapy (b)
Figure 6. MRI of femurs in coronal projection (a, b: TT-WIj. Bone marrow infiltration by Gauche cells before treatment (aj and it’s complete regression after 5 years of treatment (b)
Figure 7. MRI of the hip joints in coronal projection (Stir mode).Edema of the bone marrow of the head and neck of the right femur
Figure 7. MRI of hip joints in coronal projection (Stirj. Bone marrow edema of head and neck right femur
At the same time, the cortical layer covering the necrotic area of the bone marrow thinned with the development of a depressed subchondral fracture.Further, there was a complete separation of the sequestrum from the epiphysis of the bone with a line of high intensity on T2-weighted and low on T1-weighted, which corresponded to the stage of fragmentation (rice.ten). The stage of outcome of cortico-medullary osteonecrosis was characterized by the predominance of deforming changes with the formation of secondary osteoarthritis and was detected in 26% of patients (Fig. 11).
Figure 8. MRI of the hip joints in coronal (a: T1-WI mode) and axial (b: T2-WI mode) projections. Cortico-medullary osteonecrosis in the head of the right femur. Sequestration of the necrotic area
Figure 8. MRI of hip joints in coronal (a: TT-WI) and transversal (b: T2-WI) projections.Cortico-medullary osteonecrosis in right femoral head. Sequestration of necrotic area
Figure 9. MRI of the femurs in coronal projection (T2-WI mode). Medullary osteonecrosis in the shaft of the femur
Figure 9. MRI of femurs in coronal projection (T2-WI). Medullary osteonecrosis in diaphysis of femurs
Figure 10. Coronal MRI of the right hip joint (Stir mode).Subchondral necrotic fragment in the femoral head
Figure 10. MRI of right hip joint in coronal projection (Stir). Subchondral necrotic fragment located in femoral head
Figure 11. MRI of the hip joints in coronal projection (T2-WI mode). Aseptic necrosis of the head of the right femur at the stage of outcome; secondary osteoarthritis of the right hip joint
Figure 11. MRI of hip joints in coronal projection (T2-WI).Osteonecrosis of right femoral head. Secondary osteoarthrosis of right hip joint
- Osteosclerosis
In 12% of cases, widespread osteonecrosis led to the formation of postinfarction bone marrow osteosclerosis, which was visualized on MRI as hypointense zones in all pulse sequences (Fig. 12).
- Osteolysis
Thinning of bone tissue in HD led to bone destruction and was accompanied by the formation of areas of osteolysis, which were radially similar to solitary bone cysts.In 7% of patients, on MRI scans, elongated oval areas were visualized, which were clearly delimited from the surrounding bone tissue by a low-intensity strip of endosteal ossification. The structure of the cavities was homogeneous or cellular-trabecular with the presence of fluid or hemorrhagic contents (Fig. 13).
The described structural changes in bones occurred both in isolation and in various combinations. In accordance with the analysis of MRI tomograms of the patients examined according to a specialized formalized protocol, the following data were obtained.
Figure 12. MRI of the femurs in coronal projection (T1-WI mode). Areas of postinfarction osteosclerosis in the diaphysis and distal metaphyses of the femur
Figure 12. MRI of femurs in coronal projection (T2-WI). Areas of postinfarction osteosclerosis in diaphysis and distal metaphysis of femurs
Figure 13. MRI of the femurs in coronal projection (T2-WI mode). Massive osteolysis site in the distal metadiaphysis of the left femur
Figure 13. MRI of femurs in coronal projection (T2-WI). Huge area of osteolysis in distal metadiaphysis of left femur
Table 2. Reversible changes (according to MRI, n = 86)
Table 2. Reversible changes (according to MRI, n = 86)
3000 areas areas59
- reversible systemic changes (95%) patients (tab.2). The combination of bone marrow infiltration with trabecular edema was found in 27% of patients.
- Irreversible changes in the osteoarticular system occurred in 83 (97%) patients (Table 3). A combination of various types of irreversible changes was found in 49 (57%) patients. The most common combinations were:
a) deformity like Erlenmeyer flasks + medullary osteonecrosis – 46 (53%) patients;
b) deformity like Erlenmeyer flasks + corticomedullary osteonecrosis + secondary osteoarthritis – 22 (26%) patients;
c) Erlenmeyer flask deformity + medullary / cortico-medullary osteonecrosis + osteosclerosis – 10 (12%) patients.
According to the analysis of MRI tomograms, 3 out of 86 patients had no pathological changes in the osteoarticular system. The rest of the patients (n = 83) were divided into 4 groups depending on the severity of damage to the osteoarticular system:
- mild bone damage – 34 (40%) patients;
- moderately severe – 26 (30%) patients;
- severe bone damage – 21 (24%) patients;
- super-severe bone damage – 2 (2%) patients.
Discussion
Analysis of the accumulated data has shown a variety of radiation semiotics of bone lesions in type I HD.Damage to the osteoarticular system is a typical manifestation of HD and ranges from asymptomatic bone marrow infiltration, osteopenia to severe osteoporosis with pathological fractures and aseptic bone necrosis, leading to the development of secondary osteoarthritis and, as a consequence, irreversible orthopedic defects [12]. The development of severe concomitant diseases (for example, osteoarticular tuberculosis) [13], life-threatening complications in the form of total destruction of bone tissue with multiple pathological fractures [14] is possible.According to the International HD Registry [7], which includes 2004 patients from 39 countries, the most common symptoms of bone involvement were Erlenmeir flask-type bone deformity (61%), bone marrow infiltration (59%), and osteopenia (50%). Medullary (35%) and cortico-medullary (34%) osteonecrosis and pathological fractures (26%) were somewhat less common.
Table 3 . Irreversible changes (according to MRI data, n = 86)
Table 3 . Irreversible changes (according to MRI, n = 86)
No. | Type of changes Type of changes | Number of patients (%) Number of patients (%) | |
---|---|---|---|
1 | Bone marrow infiltration | 82 (95) | |
5 Type | |||
5 Type | 71 (82) | ||
heterogeneous heterogeneo us | 11 (13) | ||
expressed expressed | 25 (29) | ||
Severity Severity moderate Severity moderate80002 | 43 (50) | ||
weakly expressed weakly expressed | 14 (16) | ||
Prevalence (modified DOseldor scale) 9000 score 9000 | 1 area I area | 1 (1) | |
2 areas 2 areas | 4 (5) | ||
0 | |||
90 002 4 areas 4 areas | 39 (45) | ||
5 areas 5 areas | 1 (1) | ||
6 areas 9000 areas 9000 9000 37 (43) | |||
2 | Trabecular bone marrow edema | 23 (27) |
2
28395 2839723000
Osteosclerosis
5
Osteosclerosis
Osteosclerosis
No. | Type of changes Type of changes | Number of patients (%) Number of patients (%) | |
---|---|---|---|
1 | Erlenmeyer flask deformity Erlenmeyer flask deformity | 83 (97) | |
Osteonec | Osteonec | ||
2 | medullary medullary | 46 (53) | |
cortico-medullary cortico-medullary | |||
Secondary osteoarthrosis Secondary osteoarthrosis | 22 ( 26) | ||
4 | Osteosclerosis Osteosclerosis | 10 (12) | |
5 | |||
6 | Pathological fracture Pathological fracture | 7 (8) |
The main role in assessing the severity of damage to the osteoarticular system in HD belongs to radiation diagnostics.In the present work, reversible and irreversible changes were identified based on the nature of bone damage. Reversible changes included infiltration of the bone marrow with Gaucher cells, which may be associated with the development of osteopenia and osteoporosis, as well as bone marrow edema. Irreversible: osteonecrosis, foci of osteolysis, osteosclerosis, pathological fractures and bone deformities, secondary arthropathy. Bone deformity of the Erlenmeyer flask type is irreversible, but does not have clinical and prognostic significance [3], therefore it was not considered as a criterion for the severity of damage to the osteoarticular system in HD.
To characterize the severity of damage to the osteoarticular system in HD, we developed the following criteria [15].
- Mild bone damage:
- infiltration and / or edema of the bone marrow of the femur.
- Moderately severe:
- bone marrow infiltration and / or edema of the femur;
- Areas of medullary osteonecrosis of the femurs.
- Severe bone damage:
- infiltration and / or edema of the bone marrow of the femur;
- areas of medullary osteonecrosis of the femurs;
- areas of cortico-medullary osteonecrosis of the femur with the development of secondary osteoarthritis;
- osteosclerosis;
- osteolysis;
- single pathological fractures.
- Extra severe bone damage:
- infiltration and / or edema of the bone marrow of the femur;
- areas of medullary osteonecrosis of the femurs;
- areas of cortico-medullary osteonecrosis of the femur with the development of secondary osteoarthritis;
- osteosclerosis;
- osteolysis;
- multiple pathological fractures. The above criteria show that the severity of damage to the osteoarticular system is determined mainly by the presence of irreversible changes, which, in turn, determine the severity of the course of type I HD and the quality of life of patients.
Thus, the radiation semiotics of lesions of the osteoarticular system in HD patients is quite typical and at the same time extremely variable, which is due to a variety of manifestations from mild, reversible, to extremely severe, irreversible changes. MRI is the “gold standard” of modern diagnostics of bone lesions in HD, as it allows an objective assessment of bone marrow involvement and the severity of damage to the osteoarticular system in HD patients. The high sensitivity of this method in detecting reversible changes in the skeletal system that cannot be visualized using classical radiography has been demonstrated.Early diagnosis and early therapy can prevent the development of irreversible orthopedic defects that determine the quality of life in patients with type I HD.
Possibilities of prevention and rehabilitation treatment of the consequences of perinatal lesions of the central nervous system in premature infants | Pavlyukova
1. Cheong JL, Doyle LW. Increasing rates of prematurity and epidemiology of late preterm birth.J Pediatr Child Health. 2012; 48 (9): 784-788. doi: 10.1111 / j.1440-1754.2012.02536.x.
2. Beck S, Wojdyla D, Say L, et al. The worldwide incidence of preterm birth: a systematic review of maternal mortality and morbidity. Bull World Health Organ. 2010; 88 (1): 31–38. doi: 10.2471 / BLT.08.062554.
3. Modern medico-social problems of neonatology / Ed.A.A. Baranova, G.V. Yatsyk. – M .: Pediatr; 2015. – S. 39–52.
4. Soll RF. Progress in the care of extremely preterm infants. JAMA. 2015; 314 (10): 1007-1008. doi: 10.1001 / jama.2015.10911.
5. Ballot DE, Chirwa T, Ramdin T, et al. Comparison of morbidity and mortality of very low birth weight infants in a Central Hospital in Johannesburg between 2006/2007 and 2013.BMC Pediatr. 2015; 15 (1): 20. doi: 10.1186 / s12887-015-0337-4.
6. Petrou S. The economic consequences of preterm birth during the first 10 years of life. BJOG. 2005; 112 Suppl 1: 10-15. doi: 10.1111 / j.1471-0528.2005.00577.x.
7. Vinogradova I.V. Morbidity and mortality in children with extremely low body weight // Bulletin of the Chuvash University.- 2012. – No. 3 – P. 335–341
8. Yatsyk GV, Belyaeva IA, Bombardirova EP, et al. Clinical examination of newborns and infants // Russian pediatric journal. – 2012. – No. 2 – P. 22–26.
9. Doyle LW, Roberts G, Anderson PJ; Victorian Infant Collaborative Study Group. Changing long-term outcomes for infants 500-999 g birth weight in Victoria, 1979-2005.Arch Dis Child Fetal Neonatal Ed. 2011; 96 (6): F443–447. doi: 10.1136 / adc.2010.200576.
10. Kumar P, Shankaran S, Ambalavanan N, et al. Characteristics of extremely low birth weight infant survivors with unimpaired outcomes at 30 months of age. J Perinatol. 2013; 33 (10): 800–805. doi: 10.1038 / jp.2013.71.
11. Gargus RA, Vohr BR, Tyson JE, et al.Unimpaired outcomes for extremely low birth weight infants at 18 to 22 months. Pediatrics. 2009; 124 (1): 112-121. doi: 10.1542 / peds.2008-2742.
12. Vlasyuk V.V. Birth trauma and perinatal cerebrovascular accidents. – SPb .: Nestor-History; 2009 .– 252 p.
13. Barashnev Yu.I., Rozanov A.V., Panov V.O., Volobuev A.I. The role of hypoxic-traumatic brain injury in the formation of disability from childhood // Russian Bulletin of Perinatology and Pediatrics.- 2006. – T.51. – No. 4 – P. 41–46.
14. Volpe JJ. The encephalopathy of prematurity – brain injury and impaired brain development inextricably intertwined. Semin Pediatr Neurol. 2009; 16 (4): 167-178. doi: 10.1016 / j.spen.2009.09.005.
15. Namazova-Baranova LS, Deev IA, Kobyakova OS, et al. Features of somatic pathology in children with low, very low and extremely low birth weight in different age periods of life // Bulletin of Siberian Medicine.- 2016. – T.15. – No. 4 – P. 140-149. doi: 10.20538 / 1682-0363-2016-4-140-149.
16. Himpens E, Van den Broeck C, Oostra A, et al. Prevalence, type, distribution, and severity of cerebral palsy in relation to gestational age: a meta-analytic review. Dev Med Child Neurol. 2008; 50 (5): 334-340. doi: 10.1111 / j.1469-8749.2008.02047.x.
17.Department of Analysis, Forecast and Innovative Development of Health Care, Central Research Institute for Organization and Informatization of Health Care. Statistical materials on the incidence of the child population in Russia. Ministry of Health of the Russian Federation. – M .; 2012.
18. Altunin VV, Antonov AG, Baranov AA, et al. Modern approaches to the prevention, diagnosis and treatment of bronchopulmonary dysplasia.A guide for medical practitioners. – M .: Pediatr; 2013 .– 176 p.
19. Davydova I.V. Formation, course and outcomes of bronchopulmonary dysplasia in children: Author’s abstract. dis. … doct. honey. sciences. – M .; 2010 .– 48 p.
20. Principles of staged nursing of premature babies / Ed. L.S. Namazova-Baranova. – M .: Pediatr; 2013. – S. 85–94.
21. Yang I., Rosenberg G.A. Damage to the blood-brain barrier in acute and chronic cerebrovascular diseases // Stroke. – 2012. – No. 1 – P. 91–96.
22. Fan X, Kavelaars A, Heijnen CJ, et al. Pharmacological neuroprotection after perinatal hypoxic-ischemic brain injury. Curr Neuropharmacol. 2010; 8 (4): 324–334. doi: 10.2174/157015
3358150.
23. Finger A.B. The state of the nervous system of newborns. Guidelines. – SPb .: SPBGPMA; 2004 .– 22 p.
24. Order of the Ministry of Health No. 921n dated November 15, 2012 “On approval of the Procedure for the provision of medical care in the neonatology profile”.
25.Palchik A.B., Fedorova L.A. Periventricular leukomalacia. Guidelines. – SPb .: SPBGPMA; 2008 .– 45 p.
26. Palchik A.B. Shabalov N.P. Hypoxic-ischemic encephalopathy of newborns. – M .: MEDpress-inform; 2013 .– 288 p.
27. Ignatko I.V., Rybin M.V., Kalinina E.M. Pathogenesis of perinatal disorders in high-risk pregnancy // Questions of practical pediatrics.- 2006. – No. 4 – P. 27.
28. Goncharova OV, Bakanov MI, Greshilov AA, et al. Modern biochemical criteria for the diagnosis of perinatal hypoxic lesions of the central nervous system in newborns // Russian Pediatric Journal. – 2007. – No. 4 – P. 13-18.
29. de Vries LS, Benders MJ, Groenendaal F. Progress in neonatal neurology with a focus on neuroimaging in the preterm infant.Neuropediatrics. 2015; 46 (4): 234–241. doi: 10.1055 / s-0035-1554102.
30. Wezel-Meijler Gv, de Vries LS. Cranial ultrasound – optimizing utility in the NICU. Curr Pediatr Rev. 2014; 10 (1): 16–27. doi: 10.217 4/157339631001140408120106.
31. Panigrahy A, Wisnowski JL, Furtado A, et al. Neuroimaging bio-markers of preterm brain injury: toward developing the preterm con-nectome.Pediatr Radiol. 2012; 42 Suppl 1: S33–61. doi: 10.1007 / s00247-011-2239-4.
32. Stoll BJ, Hansen NI, Bell EF, et al. Neonatal outcomes of extremely preterm infants from the NICHD Neonatal Research Network. Pediatrics. 2010; 126 (3): 443–456. doi: 10.1542 / peds.2009-2959.
33. Harteman JC, Groenendaal F, van Haastert IC, et al. Atypical timing and presentation of periventricular haemorrhagic infarction in preterm infants: the role of thrombophilia.Dev Med Child Neurol. 2012; 54 (2): 140-147. doi: 10.1111 / j.1469-8749.2011.04135.x.
34. Vasilyuk V.V. Clinical and morphological classification of intraventricular hemorrhages in the brain // Questions of modern pediatrics. – 2013. – T.12. – No. 6 – P. 48–52. doi: 10.15690 / vsp.v12i6.873.
35. Odinak M.M., Tsygan N.V. Growth factors of nervous tissue in the central nervous system.- SPb .: Science; 2005 .– 157 p.
36. Marro PJ. The etiology and pharmacologic approach to hypoxic-ischemic encephalopathy in the newborn. Neoreviews. 2007; 3 (6): e99-107. doi: 10.1542 / neo.3-6-e99.
37. Bassan H, Limperopoulos C, Visconti K, et al. Neurodevelpmental outcome in survivors of periventricular hemorrhagic infarction. Pediatrics.2007; 120 (4): 785-792. doi: 10.1542 / peds.2007-0211.
38. Walgen NG, Ahmed NA. Neuroprotection in cerebral ishaemia: facts and fancies-the need for new approaches. Cerebrovasc Dis. 2004; 17 Suppl 1: 153-166. doi: 10.1159 / 000074808.
39. Fukuda S, Kato T, Kakita H, et al. Hemodynamics of cerebral arteries of infants with periventricular leukomalcia.Pediatrics. 2006; 117 (1): 1-8. doi: 10.1542 / peds.2004-1719.
40. Neurobiological foundations of the emergence and rehabilitation treatment of perinatal CNS lesions in children / Ed. L.S. Namazova-Baranova. – M .: Pediatr; 2016 .– 184 p.
41. Zakharova L.I., Tupikova S.A. Possibilities of neuroprotective therapy in perinatology // Ulyanovsk medical and biological journal.-2017. – No. 3 – C 62–71. doi: 10.23648 / UMBJ.2017.27.7077.
42. Filchenkov A.A. Caspases: regulators of apoptosis and other cellular functions // Biochemistry. – 2003. – T.68. – No. 4 – P. 453–466.
43. Chamnanvanakij S, Margraf LR, Burns D, Perlman JM. Apoptosis and white matter injury in preterm infants. Pediatr Dev Pathol. 2002; 5 (2): 184-189.doi: 10.1007 / s10024-001-0205-0.
44. Gomazkov O.A. Neurogenesis as an adaptive function of the brain. – M .: Ikar; 2013 .– 136 p.
45. Austin T, O’Reilly H. Advances in imaging the neonatal brain. Expert Opin Med Diagn. 2011; 5 (2): 95-107. doi: 10.1517 / 17530059.2011.554819.
46.Kwon SH, Vasung L, Ment LR, Huppi PS. The role neuroimaging in predicting neurodevelopmental outcomes of preterm neonates. Clin Perinatol. 2014; 41 (1): 257-283. doi: 10.1016 / j.clp.2013.10.003.
47. Nosarti C, Walshe M, Rushe TM, et al. Neonatal ultrasound results following very preterm birth predict adolescent behavioral and cognitive outcome. Dev Neuropsychol. 2011; 36 (1): 118-135. doi: 10.1080 / 87565641.2011.540546.
48. Krivtsova L.A., Belsky V.V. Methods of neuroimaging in predicting the outcomes of cerebral ischemia in children of the first year of life // Bulletin of new medical technologies – 2013. – T. XX. – No. 2 – P.423–427.
49. van Wezel-Meijler G, Steggerda SJ, Leijser LM. Cranial ultra-sonography in neonates: role and limitatio ns. Semin Perinatol.2010; 34 (1): 28–38. doi: 10.1053 / j.semperi.2009.10.002.
50. Freeman JM. The use of amplitude-integrated electroencephalography: beware of its unintended consequences. Pediatrics. 2011; 119 (3): 615-617. doi: 10.1542 / peds.2006-3650.
51. Veselova A.N., Vatolin K.V. Principles of diagnosis and treatment of cerebral blood flow disorders in hypoxic-ischemic lesions of the central nervous system in newborn children // Bulletin of Pediatric Pharmacology and Nutrition.- 2007. – T.4. – No. 4 – P. 51–55.
52. V. N. Timoshenko. Premature newborn babies. Tutorial. – Rostov-on-Don; 2007 .– 184 p.
53. Dyusenova SB, Korneeva EA, Dombrovskaya IL Consequences of posthypoxic changes in the brain in children: clinical features and diagnostics // Successes of modern natural science. – 2014.- No. 7 – P. 9-11.
54. Beaino G, Khoshnood B, Kaminski M, et al. Predictors of cerebral palsy in very preterm infants: the EPIPAGE prospective population-based cohort study. Dev Med Child Neurol. 2010; 52 (6): e119-125. doi: 10.1111 / j.1469-8749.2010.03612.x.
55. Nosarti C. Neurodevelopmental outcomes of preterm birth: from childhood to adult life.Nosarti C, Murray R, Hack M, editors. Cambridge: University Press; 2010. pp. 39-53.
56. Edwards AD, Arthurs OJ. Paediatric MRI under sedation: is it necessary? What is the evidence for the alternatives? Pediatr Radiol. 2011; 41 (11): 1353-1364. doi: 10.1007 / s00247-011-2147-7.
57. Hillenbrand CM, Reykowski A. MR imaging of the newborn: a technical perspective.Magn Reson Imaging Clin N Am. 2012; 20 (1): 63–79. doi: 10.1016 / j.mric.2011.08.010.
58. Arthurs OJ, Edwards A, Austin T, et al. The challenges of neonatal magnetic resonance. Pediatr Radiol. 2012; 42 (10): 1183-1194. doi: 10.1007 / s00247-012-2430-2.
59. Neubauer V, Griesmaier E, Baumgartner K, et al. Feasibility of cerebral MRI in non-sedated preterm-born infants at term-equivalent age: report of a single center.Acta Paediatr. 2011; 100 (12): 1544-1547.doi: 10.1111 / j.1651-2227.2011.02388.x.
60. Mathur A, Neil JJ, Inder TE. Understanding brain injury and neurodevelopmental disabilities in the preterm infant: the evolving role of advanced MRI. Semin Perinatol. 2010; 34 (1): 57–66. doi: 10.1053 / j.semperi.2009.10.006.
61. Mamedyarov A.M., Namazova-Baranova L.S., Eromlina Yu.V., et al. Possibilities of assessing motor and sensory pathways of the brain using diffusion tensor tractography in children with infantile cerebral palsy // Bulletin of the Russian Academy of Medical Sciences. – 2014. – T.69. – No. 9-10 – P. 70-76. doi: 10.15690 / vramn.v69i9-10.1134.
62. Tusor N, Arichi T, Counsell SJ, et al. Brain development in preterm infants assessed using advanced MRI techniques.Clin Perinatol. 2014; 41 (1): 25–45. doi: 10.1016 / j.clp.2013.10.001.
63. Doria V, Arichi T, Edwards DA. Magnetic resonance imaging of the preterm infant brain. Curr Pediatr Rev. 2014; 10 (1): 48–55. doi: 10.2 174/157339631001140408120821.
64. Smyser C, Kidokoro H, Inder T. MRI of the brain at term equivalent age in extremely premature neonates – to scan or not to scan? J Paediatr Child Health.2012; 48 (9): 794-800. doi: 10.1111 / j.1440-1754.2012.02535.x.
65. Hoon AH Jr, Vasconcellos Faria A. Pathogenesis, neuroimaging and management in children with cerebral palsy born preterm. Dev Disabil Res Rev. 2010; 16 (4): 302-312. doi: 10.1002 / ddrr.127.
66. Ermolina Yu.V., Namazova-Baranova L.S., Mamedyarov A.M., et al. The role of diffusion tensor magnetic resonance imaging and tractography in the diagnosis of structural brain damage in children with cerebral palsy // Questions of modern pediatrics.- 2016. – T.15. – No. 2 – P. 141-147. doi: 10.15690 / vsp.v15i2.1531.
67. Yermolina Yu.V. Features of structural and functional changes in the brain in children with cerebral palsy: Author’s abstract. dis. … Cand. honey. sciences. – M .; 2016 .– 24 p.
68. Order of the Government of the Russian Federation of August 31, 2016 No. 1839-r. Available at: https: // government.consultant.ru/ documents / 3712398. Link active on 01/12/2018.
69. Baranov A.A., Albitskiy V.Yu., Terletskaya R.N., Zelinskaya D.I. Multilevel system of providing medical care to the child population // Questions of modern pediatrics. – 2014. – T.13. – No. 2 – P. 5-10.
70. Vygotsky, L.S. Collected works in six volumes. – T.2, T.5. – M .: Eksmo; 2005 .– 1136 p.
71. Psychological and pedagogical diagnostics of the development of children of early and preschool age. Methodical manual / Ed. E.A. Strebeleva. – M .: Education; 2005.
72. Diagnostics and complex rehabilitation of perinatal pathology of newborns / Ed. G.V. Yatsyk. – M .: Pediatr; 2012 .– 156 p.
73. Lazurenko S.B. Mental development of children with health disorders at an early age. – M .: Logomag; 2014 .– 266 p.
74. Lazurenko S.B. Organization of correctional and pedagogical assistance to children with central nervous system damage in health care institutions (methodological letter). – M: FGUP TsBNTI Roszdrav; 2008 .– 60 p.
75.Principles of staged nursing of premature babies / Ed. L.S. Namazova-Baranova. – M .: Pediatr; 2013. – S. 171–237.
76. Zavidova S.S., Namazova-Baranova L.S., Topolyanskaya S.V. Clinical trials of drugs in pediatrics: problems and achievements // Pediatric Pharmacology. – 2010. – T.7. – No. 1 – P. 6-14.
77.Tatochenko V.K. Vaccination of premature and low birth weight babies // Pediatric Pharmacology. – 2013. – T.10. – No. 4 – P. 30–36. doi: 10.15690 / pf.v10i4.752.
78. Kazakova KA, Namazova-Baranova LS, Davydova IV, et al. Primary serological status and immunological efficacy of vaccination against Streptococcus pneumoniae and Haemophilus influenza type b in children with bronchopulmonary dysplasia: a cohort study / / Pediatric Pharmacology – 2018.- T.15. – No. 1 – P. 43–49. doi: 10.15690 / pf.v15i1.1842.
79. Zubova E.P., Farrakhov A.Z., Shavaliev R.F., Sadykov M.M. Provision of outpatient care for children with developmental disabilities at an early age // Medical Almanac. – 2014. —No. 1 – P. 10-14.
80. Polunina VV, Suyundukova AS, Sergeenko E.Yu., et al. Rehabilitation of children with disabilities in health on an outpatient basis // Questions of modern pediatrics.- 2011. – T.10. – No. 6 – P. 14-19.
81. Yanovskaya N.V., Evtushenko O.S., Evtushenko S.K. Methodology for the rehabilitation of children 1 year of life with delayed motor and pre-speech development (threatened by the development of cerebral palsy) in the context of specialized inpatient treatment of the department of the Donetsk Regional Clinical Center for Neurorehabilitation // International Neurological Journal. – 2014. – No. 3 – P. 177–178.
82.Belyaeva I.A., Bombardirova E.P., Tokovaya E.I., et al. Non-drug habilitation of children with perinatal lesions of the nervous system // Questions of modern pediatrics. – 2017. – T.16. – No. 5 – P. 383–391. doi: 10.15690 / vsp.v16i5.1802.
.